A Tour of the Cell: Crash Course Biology #23
< Back to Crash Course Series
The cell is the basic unit of life, and our understanding of it has advanced as science, and the tools available to scientists, has advanced. In this episode of Crash Course Biology, we’ll take a look at the difference between prokaryotic and eukaryotic cells, take a guided tour of the eukaryotic cell, and learn why most cells are small. We’ll explore the eukaryotic cell’s surprising beginnings through an endosymbiosis that occurred about 1.5 billion years ago.
Spanish Video

Related BioInteractive Resources
- Cystic Fibrosis Mechanism and Treatment
- Root Movement
Sciencing_Icons_Science SCIENCE
Sciencing_icons_biology biology, sciencing_icons_cells cells, sciencing_icons_molecular molecular, sciencing_icons_microorganisms microorganisms, sciencing_icons_genetics genetics, sciencing_icons_human body human body, sciencing_icons_ecology ecology, sciencing_icons_chemistry chemistry, sciencing_icons_atomic & molecular structure atomic & molecular structure, sciencing_icons_bonds bonds, sciencing_icons_reactions reactions, sciencing_icons_stoichiometry stoichiometry, sciencing_icons_solutions solutions, sciencing_icons_acids & bases acids & bases, sciencing_icons_thermodynamics thermodynamics, sciencing_icons_organic chemistry organic chemistry, sciencing_icons_physics physics, sciencing_icons_fundamentals-physics fundamentals, sciencing_icons_electronics electronics, sciencing_icons_waves waves, sciencing_icons_energy energy, sciencing_icons_fluid fluid, sciencing_icons_astronomy astronomy, sciencing_icons_geology geology, sciencing_icons_fundamentals-geology fundamentals, sciencing_icons_minerals & rocks minerals & rocks, sciencing_icons_earth scructure earth structure, sciencing_icons_fossils fossils, sciencing_icons_natural disasters natural disasters, sciencing_icons_nature nature, sciencing_icons_ecosystems ecosystems, sciencing_icons_environment environment, sciencing_icons_insects insects, sciencing_icons_plants & mushrooms plants & mushrooms, sciencing_icons_animals animals, sciencing_icons_math math, sciencing_icons_arithmetic arithmetic, sciencing_icons_addition & subtraction addition & subtraction, sciencing_icons_multiplication & division multiplication & division, sciencing_icons_decimals decimals, sciencing_icons_fractions fractions, sciencing_icons_conversions conversions, sciencing_icons_algebra algebra, sciencing_icons_working with units working with units, sciencing_icons_equations & expressions equations & expressions, sciencing_icons_ratios & proportions ratios & proportions, sciencing_icons_inequalities inequalities, sciencing_icons_exponents & logarithms exponents & logarithms, sciencing_icons_factorization factorization, sciencing_icons_functions functions, sciencing_icons_linear equations linear equations, sciencing_icons_graphs graphs, sciencing_icons_quadratics quadratics, sciencing_icons_polynomials polynomials, sciencing_icons_geometry geometry, sciencing_icons_fundamentals-geometry fundamentals, sciencing_icons_cartesian cartesian, sciencing_icons_circles circles, sciencing_icons_solids solids, sciencing_icons_trigonometry trigonometry, sciencing_icons_probability-statistics probability & statistics, sciencing_icons_mean-median-mode mean/median/mode, sciencing_icons_independent-dependent variables independent/dependent variables, sciencing_icons_deviation deviation, sciencing_icons_correlation correlation, sciencing_icons_sampling sampling, sciencing_icons_distributions distributions, sciencing_icons_probability probability, sciencing_icons_calculus calculus, sciencing_icons_differentiation-integration differentiation/integration, sciencing_icons_application application, sciencing_icons_projects projects, sciencing_icons_news news.
- Share Tweet Email Print
Eukaryotic Cells
Prokaryotic cells, cell growth & division, cellular metabolism, cell physiology & behavior, epithelial cells, specialized cell types.
- Home ⋅
- Science ⋅
- Biology ⋅
- Cell (Biology): An Overview of Prokaryotic & Eukaryotic Cells
Eukaryotic Cell: Definition, Structure & Function (with Analogy & Diagram)
As you've learned already, cells are the basic unit of life.
And whether you're hoping to ace your middle school or high school biology tests or are looking for a refresher before college biology, knowledge eukaryotic cell structure is a must-have.
Read on for a general overview that'll cover everything you need to know for (most) middle school and high school biology courses. Follow the links for detailed guides to each cell organelle to ace your courses.
Overview of Eukaryotic Cells
What exactly are eukaryotic cells? They're one of two major classifications of cells – eukaryotic and prokaryotic . They're also the more complex of the two. Eukaryotic cells include animal cells – including human cells – plant cells, fungal cells and algae.
Eukaryotic cells are characterized by a membrane-bound nucleus. That's distinct from prokaryotic cells, which have a nucleoid – a region that's dense with cellular DNA – but don't actually have a separate membrane-bound compartment like the nucleus.
Eukaryotic cells also have organelles, which are membrane-bound structures found within the cell. If you looked at eukaryotic cells under a microscope, you'd see distinct structures of all shapes and sizes. Prokaryotic cells, on the other hand, would look more uniform because they don't have those membrane-bound structures to break up the cell.
So why do organelles make eukaryotic cells special?
Think of organelles like rooms in your home: your living room, bedrooms, bathrooms and so on. They're all separated by walls – in the cell, these would be the cell membranes – and each type of room has its own distinct use that, overall, make your home a comfy place to live. Organelles work a similar way; they all have distinct roles that help your cells function.
All those organelles help eukaryotic cells carry out more complex functions. So, organisms with eukaryotic cells – like humans – are more complex than prokaryotic organisms, like bacteria.
The Nucleus: The Control Center of the Cell
Let's chat about the the "brain" of the cell: the nucleus , which holds most of the cell's genetic material. Most of your cell's DNA is located in the nucleus, organized into chromosomes. In humans, that means 23 pairs of two chromosomes, or 26 chromosomes overall.
The nucleus is where your cell makes decisions about which genes will be more active (or "expressed") and which genes will be less active (or "suppressed"). It's the site of transcription, which is the first step toward protein synthesis and expressing a gene into a protein.
The nucleus is surrounded by a bilayer nuclear membrane called the nuclear envelope. The envelope contains several nuclear pores, which allow substances, including genetic material and messenger RNA or mRNA , to pass into and out of the nucleus.
And, finally, the nucleus houses the nucleolus, which is the largest structure in the nucleus. The nucleolus helps your cells produce ribosomes – more on those in a second – and also plays a role in the cell's stress response.
The Cytoplasm
In cell biology, each eukaryotic cell is separated into two categories: the nucleus, which we just described above, and the cytoplasm, which is, well, everything else.
The cytoplasm in eukaryotic cells contains the other membrane-bound organelles we'll discuss below. It also contains a gel-like substance called cytosol – a mix of water, dissolved substances and structural proteins – that makes up about 70 percent of the cell's volume.
The Plasma Membrane: The Outer Boundary
Every eukaryotic cell – animal cells, plant cells, you name it – is enveloped by a plasma membrane. The plasma membrane structure is made up of several components, depending on the type of cell you're looking at, but they all share one major component: a phospholipid bilayer .
Each phospholipid molecule is made up of a hydrophilic (or water-loving) phosphate head, plus two hydrophobic (or water-hating) fatty acids. The double membrane forms when two layers of phospholipids line up tail to tail, with the fatty acids forming the inner layer of the membrane and the phosphate groups on the outside.
This arrangement creates distinct borders for the cell, making each eukaryotic cell its own distinct unit.
There are other components of the plasma membrane, too. Proteins within the plasma membrane help transport materials in and out of the cell, and they also receive chemical signals from the environment to which your cells can react.
Some of the proteins in the plasma membrane (a group called glycoproteins ) also have carbohydrates attached. Glycoproteins act as "identification" for your cells, and they play an important role in immunity.
The Cytoskeleton: The Cellular Support
If a cell membrane doesn't sound all that strong and secure, you're right – it's not! So your cells need a cytoskeleton underneath to help maintain the cell's shape. The cytoskeleton is made up of structural proteins that are strong enough to support the cell, and that can even help the cell grow and move.
There are three major types of filaments that make up the eukaryotic cell cytoskeleton:
- Microtubules : These are the largest filaments in the cytoskeleton, and they're made of a protein called tubulin. They're extremely strong and resistant to compression, so they're key to keeping your cells in the proper shape. They also play a role in cell motility or mobility , and they also help transport material within the cell.
- Intermediate filaments: These medium-sized filaments are made of keratin (which, FYI, is also the main protein found in your skin, nails and hair). They work together with the microtubules to help maintain the cell's shape.
- Microfilaments: The smallest class of filaments in the cytoskeleton, microfilaments are made of a protein called actin . Actin is highly dynamic – actin fibers can easily get shorter or longer, depending what your cell needs. Actin filaments are especially important for cytokinesis (when one cell splits into two at the end of mitosis) and also plays a key role in cell transport and mobility.
The cytoskeleton is the reason eukaryotic cells can take on very complex shapes ( check out this crazy nerve shape! ) without, well, collapsing in on themselves.
The Centrosome
Look at an animal cell on the microscope and you'll find another organelle, the centrosome , that's closely related to the cytoskeleton.
The centrosome functions as the main microtubule organizing center (or MTOC) of the cell. The centrosome plays a crucial role in mitosis – so much that defects in the centrosome are linked to cell growth diseases, like cancer.
You'll find the centrosome only in animal cells. Plant and fungal cells use different mechanisms to organize their microtubules.
The Cell Wall: The Protector
While all eukaryotic cells contain a cytoskeleton, some types of cells – like plant cells – have a cell wall for even more protection. Unlike the cell membrane, which is relatively fluid, the cell wall is a rigid structure that helps maintain the shape of the cell.
The exact makeup of the cell wall depends on what type of organism you're looking at (algae, fungi and plant cells all have distinct cell walls). But they're generally made of polysaccharides , which are complex carbohydrates, as well as structural proteins for support.
The plant cell wall is part of what helps plants stand up straight (at least, until they're so deprived of water that they start to wilt) and stand up to environmental factors like wind. It also functions as a semi-permeable membrane, allowing certain substances to pass into and out of the cell.
The Endoplasmic Reticulum: The Manufacturer
Those ribosomes produced in the nucleolus?
You'll find a bunch of 'em in the endoplasmic reticulum, or ER . Specifically you'll find them in the rough endoplasmic reticulum (or RER), which gets its name from the "rough" appearance it has thanks to all those ribosomes.
In general, the ER is the manufacturing plant of the cell, and it's responsible for producing substances your cells need to grow. In the RER, ribosomes work hard to help your cells produce the thousands and thousands of different proteins that your cells need to survive.
There's also a portion of the ER not covered with ribosomes, called the smooth endoplasmic reticulum (or SER). The SER helps your cells produce lipids, including the lipids that form the plasma membrane and organelle membranes. It also helps produce certain hormones, like estrogen and testosterone.
The Golgi Apparatus: The Packing Plant
While the ER is the manufacturing plant of the cell, the Golgi apparatus , sometimes called Golgi body, is the packing plant of the cell.
The Golgi apparatus takes proteins newly produced in the ER and "packages" them so they can function properly in the cell. It also packages substances into small membrane-bound units called vesicles, and then they're shipped off to their proper place in the cell.
The Golgi apparatus is made up of small sacs called cisternae (they look like a stack of pancakes under a microscope) that help process materials. The cis face of the golgi apparatus is the incoming side that accepts new materials, and the trans face is the outgoing side that releases them.
Lysosomes: The "Stomachs" of the Cell
Lysosomes also play a key role in processing proteins, fats and other substances. They're small, membrane-bound organelles, and they're highly acidic, which helps them function like the "stomach" of your cell.
The lysosomes' job is to digest materials, breaking down unwanted proteins, carbohydrates and lipids so they can be removed from the cell. Lysosomes are an especially important part of your immune cells because they can digest pathogens – and keep them from harming you overall.
The Mitochondria: The Powerhouse
So where does your cell get the energy for all that manufacturing and shipping? The mitochondria , sometimes called the powerhouse or battery of the cell. The singular of mitochondria is mitochondrion.
As you've probably guessed, the mitochondria are the main sites of energy production. Specifically, they're where the last two phases of cellular respiration take place – and the location where the cell produces most of its usable energy, in the form of ATP .
Like most organelles, they're surrounded by a lipid bilayer. But the mitochondria actually have two membranes (an inner and outer membrane). The inner membrane is closely folded in on itself for more surface area, which gives each mitochondrion more space to carry out chemical reactions and produce more fuel for the cell.
Different cell types have different numbers of mitochondria. Liver and muscle cells, for instance, are particularly rich in them.
Peroxisomes
While the mitochondria might be the powerhouse of the cell, the peroxisome is a central part of the cell's metabolism.
That's because peroxisomes help absorb nutrients within your cells and come packed with digestive enzymes to break them down. Peroxisomes also contain and neutralize hydrogen peroxide – which could otherwise harm your DNA or cell membranes – to promote the long-term health of your cells.
The Chloroplast: The Greenhouse
Not every cell contains chloroplasts – they're not found in plant or fungal cells, but they are found in plant cells and some algae – but those that do put them to good use. Chloroplasts are the site of photosynthesis, the set of chemical reactions that help some organisms produce usable energy from sunlight and also help remove carbon dioxide from the atmosphere.
Chloroplasts are packed with green pigments called chlorophyll, which capture certain wavelengths of light and set off the chemical reactions that make up photosynthesis. Look inside a chloroplast and you'll find pancake-like stacks of material called thylakoids , surrounded by open space (called the stroma ).
Each thylakoid has its own membrane – the thylakoid membrane – as well.
The Vacuole
Check out a plant cell under the microscope and you're likely to see a big bubble taking up plenty of space. That's the central vacuole.
In plants, the central vacuole fills up with water and dissolved substances, and it can become so large that it takes up three-quarters of the cell. It applies turgor pressure to the cell wall to help "inflate" the cell so that the plant can stand up straight.
Other types of eukaryotic cells, like animal cells, have smaller vacuoles. Different vacuoles help store nutrients and waste products, so they stay organized within the cell.
Plant Cells vs. Animal Cells
Need a refresher on the biggest differences between plant and animal cells ? We've got you covered:
- The vacuole : Plant cells contain at least one large vacuole to maintain the cell's shape, while animal vacuoles are smaller in size.
- The centriole : Animal cells have one; plant cells don't.
- Chloroplasts : Plant cells have them; animal cells don't.
- The cell wall : Plant cells have an outer cell wall; animal cells simply have the plasma membrane.
Related Articles
- Florida State University: Molecular Expressions: Animal Cell Structure
- University of the West Indies: Ultrastructure of the Eukaryotic Cell
- Windows to the Universe: Organelles of Eukaryotic Cells
About the Author
Sylvie Tremblay holds a Master of Science in molecular and cellular biology and has years of experience as a cancer researcher and neuroscientist. Before launching her writing business, she worked as a TA and tutored students in biology, chemistry, math and physics.

Find Your Next Great Science Fair Project! GO
- The Open University
- Guest user / Sign out
- Study with The Open University
My OpenLearn Profile
Personalise your OpenLearn profile, save your favourite content and get recognition for your learning
About this free course
Become an ou student, download this course, share this free course.

Start this free course now. Just create an account and sign in. Enrol and complete the course for a free statement of participation or digital badge if available.
4 The organisation of eukaryotic cells
In eukaryotic cells many activities are compartmentalised within the organelles. The different organelles serve different functions, although in fact each type of organelle (e.g. the nucleus, or the endoplasmic reticulum, described later in this section) may play a role in several different activities. Some activities, for example the production and processing of proteins, involve different parts of the cell, or several organelles, as you will see.
Suggest some key cellular functions or processes.
You may have thought of the synthesis of different macromolecules (DNA, proteins, lipids and carbohydrates), secretion, movement, cell division, protection, and there are many more!
Below, a 'tour' through the eukaryotic cell describes the different subcellular components and their functions, with an emphasis on a typical higher (mammalian) animal cell, but also describing some of the cell components in other eukaryotic organisms. It should become clear, as you work through this section, that eukaryotic cells and their components are highly dynamic.
AP Notes, Outlines, Study Guides, Vocabulary, Practice Exams and more!

Class Notes
Social science.
- European History
- Human Geography
- US Gov and Politics
- World History
- Trigonometry
- Environmental Science
- Art History
- Music Theory
Textbook Notes
Members only.
- Textbook Request
You are here
Chapter 06 - a tour of the cell.
Chapter 6 A Tour of the Cell Lecture Outline
Overview: The Importance of Cells
- Many organisms are single-celled.
- Even in multicellular organisms, the cell is the basic unit of structure and function.
- The cell is the simplest collection of matter that can live.
- All cells are related by their descent from earlier cells.
Concept 6.1 To study cells, biologists use microscopes and the tools of biochemistry
- The discovery and early study of cells progressed with the invention of microscopes in 1590 and their improvement in the 17th century.
- The lenses refract light such that the image is magnified into the eye or onto a video screen.
- Magnification is the ratio of an object’s image to its real size.
- It is the minimum distance two points can be separated and still be distinguished as two separate points.
- Resolution is limited by the shortest wavelength of the radiation used for imaging.
- The minimum resolution of a light microscope is about 200 nanometers (nm), the size of a small bacterium.
- At higher magnifications, the image blurs.
- Techniques developed in the 20th century have enhanced contrast and enabled particular cell components to be stained or labeled so they stand out.
- While a light microscope can resolve individual cells, it cannot resolve much of the internal anatomy, especially the organelles.
- Because resolution is inversely related to wavelength used, electron microscopes (whose electron beams have shorter wavelengths than visible light) have finer resolution.
- Theoretically, the resolution of a modern EM could reach 0.002 nanometer (nm), but the practical limit is closer to about 2 nm.
- A TEM aims an electron beam through a thin section of the specimen.
- The image is focused and magnified by electromagnets.
- To enhance contrast, the thin sections are stained with atoms of heavy metals.
- The sample surface is covered with a thin film of gold.
- The beam excites electrons on the surface of the sample.
- These secondary electrons are collected and focused on a screen.
- The result is an image of the topography of the specimen.
- The SEM has great depth of field, resulting in an image that seems three-dimensional.
- However, electron microscopes can only be used on dead cells.
- Light microscopes do not have as high a resolution, but they can be used to study live cells.
- Microscopes are major tools in cytology, the study of cell structures.
- Cytology combined with biochemistry, the study of molecules and chemical processes in metabolism, to produce modern cell biology.
Cell biologists can isolate organelles to study their functions.
- The goal of cell fractionation is to separate the major organelles of the cells so their individual functions can be studied.
- This process is driven by an ultracentrifuge, a machine that can spin at up to 130,000 revolutions per minute and apply forces of more than 1 million times gravity (1,000,000 g).
- Fractionation begins with homogenization, gently disrupting the cell.
- As the process is repeated at higher speeds and for longer durations, smaller and smaller organelles can be collected in subsequent pellets.
- Cell fractionation prepares isolates of specific cell components.
- For example, one cellular fraction was enriched in enzymes that function in cellular respiration.
- Electron microscopy revealed that this fraction is rich in mitochondria.
- This evidence helped cell biologists determine that mitochondria are the site of cellular respiration.
- Cytology and biochemistry complement each other in correlating cellular structure and function.
Concept 6.2 Eukaryotic cells have internal membranes that compartmentalize their functions
Prokaryotic and eukaryotic cells differ in size and complexity.
- All cells are surrounded by a plasma membrane.
- The semifluid substance within the membrane is the cytosol, containing the organelles.
- All cells contain chromosomes that have genes in the form of DNA.
- All cells also have ribosomes, tiny organelles that make proteins using the instructions contained in genes.
- A major difference between prokaryotic and eukaryotic cells is the location of chromosomes.
- In a eukaryotic cell, chromosomes are contained in a membrane-enclosed organelle, the nucleus.
- In a prokaryotic cell, the DNA is concentrated in the nucleoid without a membrane separating it from the rest of the cell.
- In eukaryote cells, the chromosomes are contained within a membranous nuclear envelope.
- All the material within the plasma membrane of a prokaryotic cell is cytoplasm.
- These membrane-bound organelles are absent in prokaryotes.
- Eukaryotic cells are generally much bigger than prokaryotic cells.
- At the lower limit, the smallest bacteria, mycoplasmas, are between 0.1 to 1.0 micron.
- Most bacteria are 1–10 microns in diameter.
- Eukaryotic cells are typically 10–100 microns in diameter.
- Metabolic requirements also set an upper limit to the size of a single cell.
- Smaller objects have a greater ratio of surface area to volume.
- The plasma membrane functions as a selective barrier that allows the passage of oxygen, nutrients, and wastes for the whole volume of the cell.
- The volume of cytoplasm determines the need for this exchange.
- Rates of chemical exchange across the plasma membrane may be inadequate to maintain a cell with a very large cytoplasm.
- The need for a surface sufficiently large to accommodate the volume explains the microscopic size of most cells.
- Larger organisms do not generally have larger cells than smaller organisms—simply more cells.
- Cells that exchange a lot of material with their surroundings, such as intestinal cells, may have long, thin projections from the cell surface called microvilli. Microvilli increase surface area without significantly increasing cell volume.
Internal membranes compartmentalize the functions of a eukaryotic cell.
- A eukaryotic cell has extensive and elaborate internal membranes, which partition the cell into compartments.
- These membranes also participate directly in metabolism, as many enzymes are built into membranes.
- The compartments created by membranes provide different local environments that facilitate specific metabolic functions, allowing several incompatible processes to go on simultaneously in a cell.
- The general structure of a biological membrane is a double layer of phospholipids.
- Other lipids and diverse proteins are embedded in the lipid bilayer or attached to its surface.
- Each type of membrane has a unique combination of lipids and proteins for its specific functions.
- For example, enzymes embedded in the membranes of mitochondria function in cellular respiration.
Concept 6.3 The eukaryotic cell’s genetic instructions are housed in the nucleus and carried out by the ribosomes
- Additional genes are located in mitochondria and chloroplasts.
- The nucleus averages about 5 microns in diameter.
- The two membranes of the nuclear envelope are separated by 20–40 nm.
- The envelope is perforated by pores that are about 100 nm in diameter.
- At the lip of each pore, the inner and outer membranes of the nuclear envelope are fused to form a continuous membrane.
- A protein structure called a pore complex lines each pore, regulating the passage of certain large macromolecules and particles.
- The nuclear side of the envelope is lined by the nuclear lamina, a network of protein filaments that maintains the shape of the nucleus.
- There is evidence that a framework of fibers called the nuclear matrix extends through the nuclear interior.
- Within the nucleus, the DNA and associated proteins are organized into discrete units called chromosomes, structures that carry the genetic information.
- Stained chromatin appears through light microscopes and electron microscopes as a diffuse mass.
- As the cell prepares to divide, the chromatin fibers coil up and condense, becoming thick enough to be recognized as the familiar chromosomes.
- A typical human cell has 46 chromosomes.
- A human sex cell (egg or sperm) has only 23 chromosomes.
- In the nucleolus, ribosomal RNA (rRNA) is synthesized and assembled with proteins from the cytoplasm to form ribosomal subunits.
- The subunits pass through the nuclear pores to the cytoplasm, where they combine to form ribosomes.
- The mRNA travels to the cytoplasm through the nuclear pores and combines with ribosomes to translate its genetic message into the primary structure of a specific polypeptide.
Ribosomes build a cell’s proteins.
- Cell types that synthesize large quantities of proteins (e.g., pancreas cells) have large numbers of ribosomes and prominent nucleoli.
- Some ribosomes, free ribosomes, are suspended in the cytosol and synthesize proteins that function within the cytosol.
- These synthesize proteins that are either included in membranes or exported from the cell.
- Ribosomes can shift between roles depending on the polypeptides they are synthesizing.
Concept 6.4 The endomembrane system regulates protein traffic and performs metabolic functions in the cell
- Many of the internal membranes in a eukaryotic cell are part of the endomembrane system.
- In spite of these connections, these membranes are diverse in function and structure.
- The thickness, molecular composition and types of chemical reactions carried out by proteins in a given membrane may be modified several times during a membrane’s life.
- The endomembrane system includes the nuclear envelope, endoplasmic reticulum, Golgi apparatus, lysosomes, vacuoles, and the plasma membrane.
The endoplasmic reticulum manufactures membranes and performs many other biosynthetic functions.
- The endoplasmic reticulum (ER) accounts for half the membranes in a eukaryotic cell.
- The ER includes membranous tubules and internal, fluid-filled spaces called cisternae.
- The ER membrane is continuous with the nuclear envelope, and the cisternal space of the ER is continuous with the space between the two membranes of the nuclear envelope.
- Rough ER looks rough because ribosomes (bound ribosomes) are attached to the outside, including the outside of the nuclear envelope.
- Enzymes of smooth ER synthesize lipids, including oils, phospholipids, and steroids.
- These include the sex hormones of vertebrates and adrenal steroids.
- Frequent use of these drugs leads to the proliferation of smooth ER in liver cells, increasing the rate of detoxification.
- This increases tolerance to the target and other drugs, so higher doses are required to achieve the same effect.
- Muscle cells have a specialized smooth ER that pumps calcium ions from the cytosol and stores them in its cisternal space.
- When a nerve impulse stimulates a muscle cell, calcium ions rush from the ER into the cytosol, triggering contraction.
- Enzymes then pump the calcium back, readying the cell for the next stimulation.
- As a polypeptide is synthesized on a ribosome attached to rough ER, it is threaded into the cisternal space through a pore formed by a protein complex in the ER membrane.
- As it enters the cisternal space, the new protein folds into its native conformation.
- Most secretory polypeptides are glycoproteins, proteins to which a carbohydrate is attached.
- Secretory proteins are packaged in transport vesicles that carry them to their next stage.
- Membrane-bound proteins are synthesized directly into the membrane.
- Enzymes in the rough ER also synthesize phospholipids from precursors in the cytosol.
- As the ER membrane expands, membrane can be transferred as transport vesicles to other components of the endomembrane system.
The Golgi apparatus is the shipping and receiving center for cell products.
- Many transport vesicles from the ER travel to the Golgi apparatus for modification of their contents.
- The Golgi is a center of manufacturing, warehousing, sorting, and shipping.
- The Golgi apparatus is especially extensive in cells specialized for secretion.
- The membrane of each cisterna separates its internal space from the cytosol.
- One side of the Golgi, the cis side, is located near the ER. The cis face receives material by fusing with transport vesicles from the ER.
- The other side, the trans side, buds off vesicles that travel to other sites.
- During their transit from the cis to the trans side, products from the ER are usually modified.
- The Golgi can also manufacture its own macromolecules, including pectin and other noncellulose polysaccharides.
- According to the cisternal maturation model, the cisternae of the Golgi progress from the cis to the trans face, carrying and modifying their protein cargo as they move.
- Molecular identification tags are added to products to aid in sorting.
- Products are tagged with identifiers such as phosphate groups. These act like ZIP codes on mailing labels to identify the product’s final destination.
Lysosomes are digestive compartments.
- A lysosome is a membrane-bound sac of hydrolytic enzymes that an animal cell uses to digest macromolecules.
- Lysosomal enzymes can hydrolyze proteins, fats, polysaccharides, and nucleic acids.
- Proteins in the lysosomal membrane pump hydrogen ions from the cytosol into the lumen of the lysosomes.
- Rupture of one or a few lysosomes has little impact on a cell because the lysosomal enzymes are not very active at the neutral pH of the cytosol.
- However, massive rupture of many lysosomes can destroy a cell by autodigestion.
- Lysosomal enzymes and membrane are synthesized by rough ER and then transferred to the Golgi apparatus for further modification.
- Proteins on the inner surface of the lysosomal membrane are spared by digestion by their three-dimensional conformations, which protect vulnerable bonds from hydrolysis.
- Lysosomes carry out intracellular digestion in a variety of circumstances.
- The food vacuole formed by phagocytosis fuses with a lysosome, whose enzymes digest the food.
- As the polymers are digested, monomers pass to the cytosol to become nutrients for the cell.
- This recycling, or autophagy, renews the cell.
- During autophagy, a damaged organelle or region of cytosol becomes surrounded by membrane.
- A lysosome fuses with the resulting vesicle, digesting the macromolecules and returning the organic monomers to the cytosol for reuse.
- This process plays an important role in development.
- The hands of human embryos are webbed until lysosomes digest the cells in the tissue between the fingers.
- This important process is called programmed cell death, or apoptosis.
Vacuoles have diverse functions in cell maintenance.
- Food vacuoles are formed by phagocytosis and fuse with lysosomes.
- Contractile vacuoles, found in freshwater protists, pump excess water out of the cell to maintain the appropriate concentration of salts.
- The membrane surrounding the central vacuole, the tonoplast, is selective in its transport of solutes into the central vacuole.
- The functions of the central vacuole include stockpiling proteins or inorganic ions, disposing of metabolic byproducts, holding pigments, and storing defensive compounds that defend the plant against herbivores.
- Because of the large vacuole, the cytosol occupies only a thin layer between the plasma membrane and the tonoplast. The presence of a large vacuole increases surface area to volume ratio for the cell.
Concept 6.5 Mitochondria and chloroplasts change energy from one form to another
- Mitochondria and chloroplasts are the organelles that convert energy to forms that cells can use for work.
- Mitochondria are the sites of cellular respiration, generating ATP from the catabolism of sugars, fats, and other fuels in the presence of oxygen.
- They convert solar energy to chemical energy and synthesize new organic compounds such as sugars from CO2 and H2O.
- In contrast to organelles of the endomembrane system, each mitochondrion or chloroplast has two membranes separating the innermost space from the cytosol.
- Their membrane proteins are not made by the ER, but rather by free ribosomes in the cytosol and by ribosomes within the organelles themselves.
- Both organelles have small quantities of DNA that direct the synthesis of the polypeptides produced by these internal ribosomes.
- Mitochondria and chloroplasts grow and reproduce as semiautonomous organelles.
- There may be one very large mitochondrion or hundreds to thousands of individual mitochondria.
- The number of mitochondria is correlated with aerobic metabolic activity.
- A typical mitochondrion is 1–10 microns long.
- Mitochondria are quite dynamic: moving, changing shape, and dividing.
- The inner membrane divides the mitochondrion into two internal compartments.
- The first is the intermembrane space, a narrow region between the inner and outer membranes.
- The inner membrane encloses the mitochondrial matrix, a fluid-filled space with DNA, ribosomes, and enzymes.
- Some of the metabolic steps of cellular respiration are catalyzed by enzymes in the matrix.
- The cristae present a large surface area for the enzymes that synthesize ATP.
- Amyloplasts are colorless plastids that store starch in roots and tubers.
- Chromoplasts store pigments for fruits and flowers.
- Chloroplasts contain the green pigment chlorophyll as well as enzymes and other molecules that function in the photosynthetic production of sugar.
- Chloroplasts measure about 2 microns × 5 microns and are found in leaves and other green organs of plants and algae.
- The contents of the chloroplast are separated from the cytosol by an envelope consisting of two membranes separated by a narrow intermembrane space.
- The stroma contains DNA, ribosomes, and enzymes.
- The thylakoids are flattened sacs that play a critical role in converting light to chemical energy. In some regions, thylakoids are stacked like poker chips into grana.
- The membranes of the chloroplast divide the chloroplast into three compartments: the intermembrane space, the stroma, and the thylakoid space.
- Their shape is plastic, and they can reproduce themselves by pinching in two.
- Mitochondria and chloroplasts are mobile and move around the cell along tracks of the cytoskeleton.
Peroxisomes generate and degrade H2O2 in performing various metabolic functions.
- An intermediate product of this process is hydrogen peroxide (H2O2), a poison.
- The peroxisome contains an enzyme that converts H2O2 to water.
- Some peroxisomes break fatty acids down to smaller molecules that are transported to mitochondria as fuel for cellular respiration.
- Peroxisomes in the liver detoxify alcohol and other harmful compounds.
- Specialized peroxisomes, glyoxysomes, convert the fatty acids in seeds to sugars, which the seedling can use as a source of energy and carbon until it is capable of photosynthesis.
- Peroxisomes are bound by a single membrane.
- They form not from the endomembrane system, but by incorporation of proteins and lipids from the cytosol.
- They split in two when they reach a certain size.
Concept 6.6 The cytoskeleton is a network of fibers that organizes structures and activities in the cell
- The cytoskeleton is a network of fibers extending throughout the cytoplasm.
The cytoskeleton provides support, motility, and regulation.
- The cytoskeleton provides mechanical support and maintains cell shape.
- The cytoskeleton provides anchorage for many organelles and cytosolic enzymes.
- The cytoskeleton is dynamic and can be dismantled in one part and reassembled in another to change the shape of the cell.
- The cytoskeleton also plays a major role in cell motility, including changes in cell location and limited movements of parts of the cell.
- Cytoskeleton elements and motor proteins work together with plasma membrane molecules to move the whole cell along fibers outside the cell.
- Motor proteins bring about movements of cilia and flagella by gripping cytoskeletal components such as microtubules and moving them past each other.
- The same mechanism causes muscle cells to contract.
- Inside the cell, vesicles can travel along “monorails” provided by the cytoskeleton.
- The cytoskeleton manipulates the plasma membrane to form food vacuoles during phagocytosis.
- Cytoplasmic streaming in plant cells is caused by the cytoskeleton.
- Recently, evidence suggests that the cytoskeleton may play a role in the regulation of biochemical activities in the cell.
- There are three main types of fibers making up the cytoskeleton: microtubules, microfilaments, and intermediate filaments.
- Microtubule fibers are constructed of the globular protein tubulin.
- Each tubulin molecule is a dimer consisting of two subunits.
- A microtubule changes in length by adding or removing tubulin dimers.
- Microtubules shape and support the cell and serve as tracks to guide motor proteins carrying organelles to their destination.
- Microtubules are also responsible for the separation of chromosomes during cell division.
- These microtubules resist compression to the cell.
- Before a cell divides, the centrioles replicate.
- Many unicellular eukaryotic organisms are propelled through water by cilia and flagella.
- For example, cilia lining the windpipe sweep mucus carrying trapped debris out of the lungs.
- They are about 0.25 microns in diameter and 2–20 microns long.
- Flagella are the same width as cilia, but 10–200 microns long.
- A flagellum has an undulatory movement that generates force in the same direction as the flagellum’s axis.
- Cilia move more like oars with alternating power and recovery strokes that generate force perpendicular to the cilium’s axis.
- Both have a core of microtubules sheathed by the plasma membrane.
- Nine doublets of microtubules are arranged in a ring around a pair at the center. This “9 + 2” pattern is found in nearly all eukaryotic cilia and flagella.
- Flexible “wheels” of proteins connect outer doublets to each other and to the two central microtubules.
- The outer doublets are also connected by motor proteins.
- The cilium or flagellum is anchored in the cell by a basal body, whose structure is identical to a centriole.
- Addition and removal of a phosphate group causes conformation changes in dynein.
- Dynein arms alternately grab, move, and release the outer microtubules.
- Protein cross-links limit sliding. As a result, the forces exerted by the dynein arms cause the doublets to curve, bending the cilium or flagellum.
- Each microfilament is built as a twisted double chain of actin subunits.
- Microfilaments can form structural networks due to their ability to branch.
- The structural role of microfilaments in the cytoskeleton is to bear tension, resisting pulling forces within the cell.
- They form a three-dimensional network just inside the plasma membrane to help support the cell’s shape, giving the cell cortex the semisolid consistency of a gel.
- In muscle cells, thousands of actin filaments are arranged parallel to one another.
- Thicker filaments composed of myosin interdigitate with the thinner actin fibers.
- Myosin molecules act as motor proteins, walking along the actin filaments to shorten the cell.
- A contracting belt of microfilaments divides the cytoplasm of animal cells during cell division.
- Microfilaments assemble into networks that convert sol to gel.
- According to a widely accepted model, filaments near the cell’s trailing edge interact with myosin, causing contraction.
- The contraction forces the interior fluid into the pseudopodium, where the actin network has been weakened.
- The pseudopodium extends until the actin reassembles into a network.
- This creates a circular flow of cytoplasm in the cell, speeding the distribution of materials within the cell.
- Intermediate filaments range in diameter from 8–12 nanometers, larger than microfilaments but smaller than microtubules.
- Intermediate filaments are specialized for bearing tension.
- Intermediate filaments are more permanent fixtures of the cytoskeleton than are the other two classes.
- They reinforce cell shape and fix organelle location.
Concept 6.7 Extracellular components and connections between cells help coordinate cellular activities
Plant cells are encased by cell walls.
- The cell wall, found in prokaryotes, fungi, and some protists, has multiple functions.
- In plants, the cell wall protects the cell, maintains its shape, and prevents excessive uptake of water.
- It also supports the plant against the force of gravity.
- The thickness and chemical composition of cell walls differs from species to species and among cell types within a plant.
- The basic design consists of microfibrils of cellulose embedded in a matrix of proteins and other polysaccharides. This is the basic design of steel-reinforced concrete or fiberglass.
- A mature cell wall consists of a primary cell wall, a middle lamella with sticky polysaccharides that holds cells together, and layers of secondary cell wall.
- Plant cell walls are perforated by channels between adjacent cells called plasmodesmata.
The extracellular matrix (ECM) of animal cells functions in support, adhesion, movement, and regulation.
- Though lacking cell walls, animal cells do have an elaborate extracellular matrix (ECM).
- The primary constituents of the extracellular matrix are glycoproteins, especially collagen fibers, embedded in a network of glycoprotein proteoglycans.
- The interconnections from the ECM to the cytoskeleton via the fibronectin-integrin link permit the integration of changes inside and outside the cell.
- Embryonic cells migrate along specific pathways by matching the orientation of their microfilaments to the “grain” of fibers in the extracellular matrix.
- This may coordinate the behavior of all the cells within a tissue.
Intercellular junctions help integrate cells into higher levels of structure and function.
- Neighboring cells in tissues, organs, or organ systems often adhere, interact, and communicate through direct physical contact.
- Water and small solutes can pass freely from cell to cell.
- In certain circumstances, proteins and RNA can be exchanged.
- Animals have 3 main types of intercellular links: tight junctions, desmosomes, and gap junctions.
- This prevents leakage of extracellular fluid.
- Intermediate filaments of keratin reinforce desmosomes.
- Special membrane proteins surround these pores.
- Ions, sugars, amino acids, and other small molecules can pass.
- In embryos, gap junctions facilitate chemical communication during development.
- A cell is a living unit greater than the sum of its parts.
- For example, macrophages use actin filaments to move and extend pseudopodia to capture their bacterial prey.
- Food vacuoles are digested by lysosomes, a product of the endomembrane system of ER and Golgi.
- The enzymes of the lysosomes and proteins of the cytoskeleton are synthesized on the ribosomes.
- The information for the proteins comes from genetic messages sent by DNA in the nucleus.
- All of these processes require energy in the form of ATP, most of which is supplied by the mitochondria.
Lecture Outline for Campbell/Reece Biology, 7th Edition, © Pearson Education, Inc. 6-1
We hope your visit has been a productive one. If you're having any problems, or would like to give some feedback, we'd love to hear from you.
For general help, questions, and suggestions, try our dedicated support forums .
If you need to contact the Course-Notes.Org web experience team, please use our contact form .
Need Notes?
While we strive to provide the most comprehensive notes for as many high school textbooks as possible, there are certainly going to be some that we miss. Drop us a note and let us know which textbooks you need. Be sure to include which edition of the textbook you are using! If we see enough demand, we'll do whatever we can to get those notes up on the site for you!
About Course-Notes.Org
- Advertising Opportunities
- Newsletter Archive
- Plagiarism Policy
- Privacy Policy
- DMCA Takedown Request
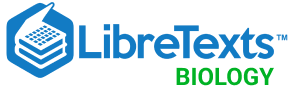
- school Campus Bookshelves
- menu_book Bookshelves
- perm_media Learning Objects
- login Login
- how_to_reg Request Instructor Account
- hub Instructor Commons
- Download Page (PDF)
- Download Full Book (PDF)
- Periodic Table
- Physics Constants
- Scientific Calculator
- Reference & Cite
- Tools expand_more
- Readability
selected template will load here
This action is not available.
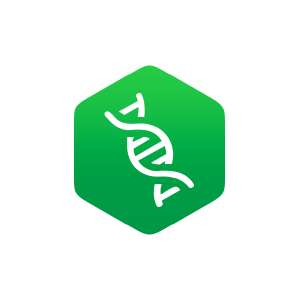
1.3: Domains of Life
- Last updated
- Save as PDF
- Page ID 88897
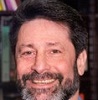
- Gerald Bergtrom
- University of Wisconsin-Milwaukee
We believe with good reason that all life on earth evolved from a common ancestral cell that existed soon after the origins of life on our planet. At one time, all life was divided into two groups: the true bacteria and everything else!
Now we group life into one of three domains :
Prokaryotes are among the first descendants of that common ancestral cell. They lack nuclei ( pro meaning before and karyon meaning kernel , or nucleus ). They include bacteria and cyanobacteria (blue-green algae).
Eukaryotes include all higher life forms, characterized by cells with true nuclei (Eu, true; karyon , nucleus )
Archaebacteria, (meaning “old” bacteria) include many extremophile bacteria (‘lovers’ of life at extreme high temperatures, salinity, etc.). Originally classified as ancient prokaryotes, Archaebacteria were shown by 1990 to be separate from prokaryotes and eukaryotes, a third domain of life.
The archaea are found in such inhospitable environments as boiling hot springs or arctic ice, though some also live in conditions that are more temperate. Based on comparison of the DNA sequences of genes for ribosomal RNAs in eukaryotes, normal bacteria (eubacteria) and extremophiles, Carl Woese proposed the three-domain phylogeny illustrated in Figure1.1:

Based on sequence similarities and differences, Woese concluded that the archaebacteria (Archaea) are not just a domain separate from the rest of the bacteria and from eukaryotes but are more closely related to eukarya than eubacteria! In fact, the Achaea share genes, proteins, and metabolic pathways found in eukaryotes but not in bacteria, supporting their close evolutionary relationship to eukaryotes. This unique sharing is further testimony to their domain status. Understanding that all living organisms belong to one of three domains has dramatically changed our understanding of evolution. Archea may be prokaryotes, but their immediate ancestors are more closely related to us than to E. coli ! For a review, see (Woese, C.2004,A new biology for anew century. Microbiol. Mol. Biol. Rev. 68:173-186) The three domains of life ( Archaea , Eubacteria and Eukarya ) quickly replaced the older division of living things into Five Kingdoms, the Monera (prokaryotes), Protista, Fungi, Plants, and Animals (all eukaryotes!)
At this point you may be asking, “What about viruses?” Where are they on, or do they even belong in the tree of life? You may already know that viruses require live cellular hosts to reproduce, but that they are not themselves alive. In fact, much about the place of viruses in evolution is an open question that we will consider in a later chapter. For now, let’s look at how we come to know about viruses and some of their peculiarities.
1.3.1. Viruses: Dead or Alive; Big or Small - A History of Surprises
Viruses that infect bacteria are called bacteriophage (phage meaning eaters, hence bacteria eaters ). Eukaryotic viruses include DNA and RNA viruses, with DNA and RNA genomes. Smallpox, hepatitis B, herpes, chicken pox/shingles, and adenovirus are caused by DNA viruses. Common colds, influenza, SARS, andCOVID-19 are caused bypositive strand RNA viruses that upon infecting a cell, replicate their RNA genome to make RNA negative strand RNAs encoding all necessary information to make new viruses. HIV AIDS, Ebola, Zika, yellow fever, and some cancers are caused by retroviruses , RNA viruses whose genome is reverse-transcribed into a cDNA intermediate that replicates and is transcribed to generate new viruses. Viruses were not identified as agents of disease until late in the nineteenth century, and we have learned much in the ensuing century. In 1892, Dmitri Ivanofsky , a Russian botanist, was studying plant diseases. One that damaged tobacco (and was therefore of agricultural significance) was themosaic disease (Figure1.2,below).
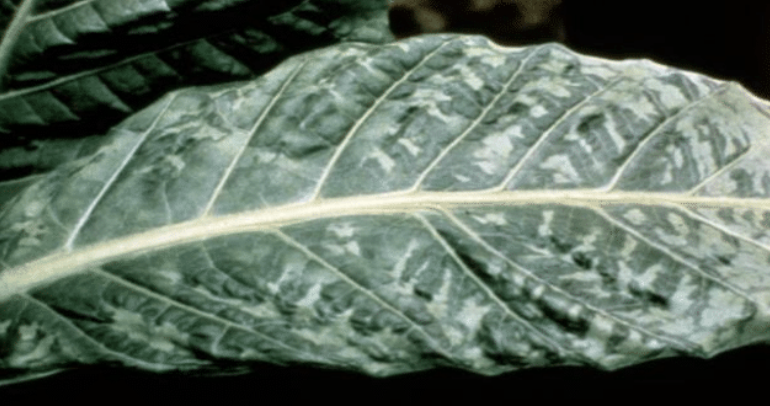
Fig 1.2 : Tobacco mosaic virus symptoms (white patches) on a tobacco leaf.
Ivanofsky showed that extracts of infected tobacco leaves were themselves infectious. The assumption was that the extracts would contain infectious bacteria.But his extracts remained infectious even after passing them through a Chamberland-Pasteur filter with a pore size so small that bacteria would not pass into the filtrate. Thus, the infectious agent(s) couldn’t be bacterial. Since the infectious material was not cellular and depended on a host for reproduction with no independent life of its own, they were soon given the name virus , a term that originally just meant toxin , or poison .This marked the start of virology , the study of viruses. The virus that Ivanofsky studied is now called Tobacco Mosaic Virus , or TMV.
Invisible by light microscopy, viruses are sub-microscopic non-cellular bits of life-chemistry that only become reproductive (come alive) when they parasitize a host cell. Since many viruses cause disease in humans, we have learned much about how they are similar and how they differ. In other chapters, we’ll learn how viruses have even become tools for the study of cell and molecular biology. Let’s start with a recent surprise from the study of viruses.
As eventually seen in the electron microscope, viruses (called virions or viral particles) are typically 150 nm or less in diameter. And that is how we have thought of viruses for over a century! But in 2002, a particle inside an amoeba, originally believed to be a bacterium, was shown by electron microscopy to be a giant virus! Since then, several more giant , or Megavirales were discovered.
Megavirales fall into two groups, pandoraviruses and mimiviruses . At 1000nm (\(1 \mu \rm m\)) Megavirus chilensis (a pandoravirus ) may be the largest. Compare a few giant viruses to a bacterium (E. coli) and the AIDS virus in Figure 1.3 below.

Consider that a typical virus contains a small genome, encoding an average of 10 genes. In contrast, the M.chilensis genome contains \(2.5 \times 10^6\) base pairs (bp) encoding up to 1,100 proteins. Still, it requires host cell proteins to infect and replicate. More surprising is that 75%of the sequenced \(1.2 \times 10^6\)bp mimivirus genome code putative proteins with no counterparts in other viruses or cells! Equally surprising, some mimiviruses genes encode proteins homologous to those used for translation in prokaryotes and eukaryotes. If all viruses, including the Megavirales , only use host cell enzymes and ribosomal machinery to synthesize proteins, what are these genes doing in a mimivirus genome? Think of the surprises here as questions. The big ones concern where and when Megavirales (giant viruses) evolved:
- What are those genes with no cellular counterparts all about?
- What were the selective advantages to a virus of large size and large genomes?
- Were Megavirales once large cells that invaded other cells, eventually becoming viral parasites and losing most but not all of their genes? Or were they once small viruses that incorporated host cell genes, increasing their genome size and coding capacity?
What information would you need (or what questions would you ask and/or what experiments could you do) to find out what the unique proteins encoded by those uniquely viral genes in e.g., mimivirus are doing for the virus?
Viruses are typically identified because they are harmful. Luc Montagnier, FrançoiseBé-Sinoussi, and Harald Z. Hausen earned the 2008 Nobel Prize in Physiology or Medicine for the discovery of HIV. Asthisis being written, the wealthy nations are emerging from theCOVID-19 pandemic caused by the SARS-CoV-2 RNA virus. Bacteria, also discovered because of the harm they do, are not only beneficial, but necessary symbionts in our many microbiomes .The book is still out on any health benefits of viruses but may someday be written!
Robert Koch discovered bacterial causes of many diseases and won the 1905 Nobel prize in Physiology or Medicine for showing that Mycobacterium tuberculosis caused tuberculosis. What other bacterial diseases did he discover and why are his four postulates still relevant?
Let’s now turn our attention to cells, entities that we define as living, with all of the properties of life ..., starting with eubacteria
1.3.2 The Prokaryotes (Eubacteria = Bacteria and Cyanobacteria )
Prokaryotic cells lack nuclei and other eukaryotic organelles, such as mitochondria, chloroplasts, endoplasmic reticulum, and assorted eukaryotic vesicles and internal membranes. Transmission and scanning electron micrographs and an illustration of rod-shaped bacteria are shown below (Figure 1.4).

Bacteria do contain bacterial microcompartments (BMCs), made up entirely of protein and not surrounded by a phospholipid membrane. These function for example, in \(\rm CO_2\) fixation to sequester toxic metabolites toxic in cells. Check out Bacterial Organelles for more information. Bacteria are typically unicellular, although a few (like some cyanobacteria) live colonial lives at least some of the time.
1.3.2.a Bacterial Reproduction
Without the compartments afforded by the internal membrane systems of eukaryotic cells, all intracellular chemistries, (reproduction and gene expression (DNA replication, transcription, translation, and all the metabolic biochemistry of life) happen in the cytoplasm. Bacterial DNA is a circular double helix that duplicates as the cell grows. While not enclosed in a nucleus, bacterial DNA is concentrated in a region of the cell called the nucleoid . When not crowded at high density, bacteria replicate their DNA throughout the life of the cell, dividing by binary fission . The result is the equal partition of duplicated bacterial chromosomes into new cells. The bacterial chromosome is basically naked DNA, unassociated with proteins.
1.3.2.b Cell Motility and the Possibility of a Cytoskeleton
Movement of cells is a response to environment. Some respond to chemicals (chemotaxis), some to light (phototaxis) or even gravity (geotaxis). Bacteria move to or from nutrients, noxious chemicals, light, dark, gravitational force, etc., by one of several mechanisms. Some use a flagellum made up largely of the bacterial protein flagellin . The main proteins of eukaryotic cellflagella and cilia are the tubulins. Together with actin and other proteins, tubulins are also part of the eukaryotic cell cytoskeleton of rods and tubes. Prokaryotes were long thought to lack similar cytoskeletal components. But two bacterial homologues of eukaryotic actin and tubulin genes were recently discovered. MreB is the actin homologue. Like actin, MreB monomers polymerize to form filaments that lie under the cell membrane of bacteria (e.g.,E. coli), helping to maintain their rod-like shape. In fact, E. coli with a mutant MreB gene is spherical..., and normally spherical bacteria lack an MreB gene! MreB was also thought to form an actin-like cortical ring that in dividing eukaryotic cells constricts to pinchoff two new cells. But this function seems to be served by the FtsZ protein that encodes a eukaryotic tubulin homologue . FtsZ polymers form filaments that are seen in a Z ring at the center of a bacterial cell during binary fission . FtsZ mutants divide, but abnormally; thus the role of FtsZ in separating bacterial cells during binary fission is not yet clear. Figure 1.5 shows a micrograph and an illustration of FtsZ in the Z rings of dividing E. coli cells.

It seems that together with flagellin, the MreB and FtsZ proteins may be part of a primitive prokaryotic cytoskeleton involved in cell structure and motility, from which our own evolved!
1.3.2.c Some Bacteria Have Internal Membranes
While bacteria lack organelles (the membrane-bound structures of eukaryotic cells), internal membranes in some bacteria form as inward extensions ( invaginations ) of plasma membrane. Some of these capture energy from sunlight (photosynthesis) or from inorganic molecules ( chemolithotrophy ). Photosynthetic vesicles called Carboxysomes (Figure 1.6) are membrane bound photosynthetic vesicles in which \(\rm CO_2\) is fixed (reduced) in cyanobacteria. Photosynthetic bacteria have less elaborate internal membrane systems.

1.3.2.d Bacterial Ribosomes Do the Same Thing as Eukaryotic Ribosomes... and Look Like Them!
Ribosomes are protein-synthesizing machines. Those of prokaryotes are smaller than those of eukaryotes but can translate eukaryotic messenger RNA (mRNA) in vitro. This is because the sequences and structures of ribosomal RNAs are shared by all species, indicating long conserved evolutionary relationships. Recall that it was ribosomal sequence similarities that revealed our closer relationship to archaea than bacteria.
The prokarya (eubacteria) are a diverse group, occupying almost every wet, dry, or hot and cold nook-and-cranny of our planet. Yet, all prokaryotic cells share structural and functional metabolic properties with each other and with archaea and eukaryotes! As we’ve seen with ribosomes, this sharing supports the common ancestry of all life.
Finally, we share not only common ancestry, but living arrangements with bacteria. There are microbiomes in our gut, on our lips, in belly buttons,and in fact all over our skin(see Our Skin Microbiome for more about that!). Gut microbiome bacteria alone number~10X more than our own cells! And microbiomes are invisible but not quiet ( The HumanMicrobiome ). Interest in our microbiomes even earned them their own The NIH Human Microbiome Project .
Your microbiome is unique and could be another ‘fingerprint’ ( Microbiomes are Fingerprints ). Why is this so? Suggest circumstances in which microbiome fingerprinting might be unreliable
1.3.3 The Archaebacteria (Archaea)
Allessandro Volta, a physicist who gave his name to the ‘volt’ (electrical potential energy), discovered methane producing bacteria (methanogens) way back in 1776! He found them living in the extreme environment at the bottom of Lago Maggiore, a lake shared by Italy and Switzerland. These unusual bacteria are chemoautotrophs that get energy from \(\rm H_2\) and \(\rm CO_2\) and generate methane gas in the process. It was not until the 1960s that Thomas Brock (at theUniversity of Wisconsin-Madison) discovered thermophilic bacteria living at temperatures approaching \(100^{\circ} C\) in Yellowstone National Park in Wyoming. Organisms living in any extreme environment were soon nicknamed extremophiles . One of the thermophilic bacteria, now called Thermus aquaticus , became the source of Taq polymerase, the heat-stable DNA polymerase that made the polymerase chain reaction (PCR), now a household name in labs around the world! Extremophile and “normal” bacteria are similar in size and shape(s) and lack nuclei.This initially suggested that most extremophiles were prokaryotes. But as Carl Woese demonstrated, it is the archaea and eukarya that share a more recent common ancestry! While some bacteria and eukaryotes can live in extreme environments, the archaea include the most diverse extremophiles. Here are some of them:
- Acidophiles grow at acidic (low) pH.
- Alkaliphiles grow at high pH.
- Halophiles require high [salt], for example, Halobacterium salinarium (Figure 1.7, below left).
- Thermophiles and hyperthermophiles live at high termperatures. Pyrolobus fumarii , a hyperthermophile, lives at \(113^{\circ}C\). Thermus aquaticus (Figure 1.8, below right) normally lives at \(70^{\circ}C\). It is noted for its role in developing the polymerase chain reaction.

Right: Scanning electron micrograph of ‘heat-loving’ Thermus aquaticus bacteria ( Figure 1.8 ).
- Methanogens produce methane.
- Barophiles grow best at high hydrostatic pressure.
- Psychrophiles grow best at temperature 15 °C or lower.
- Xerophiles grow at very low water activity (i.e.,drought or near drought conditions).
- Toxicolerants grow in the presence of high levels of damaging chemicals, for example, pools of benzene, nuclear waste.
Archaea were originally seen as oddities of life, thriving in unfriendly environments. But they include organisms living in less extreme environments, including soils, marshes, and even in the human colon. They are also abundant in the oceans where they are a major part of plankton, participating in the carbon and nitrogen cycles. In the guts of cows, humans, and other mammals, methanogens facilitate digestion, generating methane gas in the process.Infact, cows have even been cited as a major cause of global warming because of their prodigious methane emissions! On the plus side, methanogenic Archaea are being exploited to create biogas and to treat sewage. Other extremophiles are the source of enzymes that function at high temperatures or in organic solvents. As already noted, some of these have become part of the biotechnology toolbox.
1.3.4. The Eukaryotes
The volume of a typical eukaryotic cell is some 1000 times that of a typical bacterial cell. Imagine a bacterium as a 100 square foot room (the size of a small bedroom, or a large walk-in closet!) with one door. Now imagine a room 1000 times as big.That is, imagine a 100,000 square foot ‘room’. You might expect many smaller rooms inside this room for such a large space to be functional. The eukaryotic cell is a lot like that large space, with lots of interior rooms (i.e., organelles) with their own entryways and exits. In fact, eukaryotic life would not even be possible without a division of labor of eukaryotic cells among different organelles (the equivalence to the small rooms in our metaphor).
The smaller prokaryotic “room” has a much larger plasma membrane surface area-to-volume ratio than a typical eukaryotic cell. This enables required environmental chemicals to enter and quickly diffuse throughout the cytoplasm of e.g., an E. coli cell. The communication between chemicals and structures in a small cell is therefore rapid. In contrast, the communication over a larger expanse of cytoplasm inside a eukaryotic cell requires the coordinated (not to mention regulated!) activities of subcellular components and compartments. Such communication can be relatively slow in a large space. In fact, eukaryotic cells have lower rates of metabolism, growth, and reproduction than prokaryotic cells. Thus, the existence of large cells required the evolution of divided labors supported by compartmentalization .
Fungi, more closely related to animal than plant cells, are a curious beast for several reasons! For one thing, the organization of fungi and fungal cells is somewhat less defined than animal cells. Structures between cells called septa separate fungal hyphae , allow passage of cytoplasm and even organelles between cells. Some primitive fungi have few or no septa, in effect creating coenocytes , which are single giant cell with multiple nuclei. Fungal cells are surrounded by a wall,whose principal component is chitin . Chitin is the same material that makes up the exoskeleton of arthropods (which includes insects and lobsters!). Typical animal and plant cells with organelles and other structures are illustrated below, in Figure 1.9 and in Figure 1.10)

We end this look at the domains of life by noting that, while eukaryotes are a tiny minority of all living species, “their collective worldwide biomass is estimated to be equal to that of prokaryotes” (Wikipedia). And we already noted that the bacteria living commensally with us humans represent 10 times as many cells as our own human cells! Clearly, each of us (and probably most animals and even plants) owes our existence to its microbiome as much we do to our own human cells. For now, keeping in mind that plants and animal cells share many internal structures and organelles that perform the same or similar functions, let’s look at them and briefly describe their functions.
Scientists discover once-in-a-billion-year event — 2 lifeforms merging to create a new cell part
Researchers think a microbe that was engulfed by an algal cell 100 million years ago has since evolved into an integral part of the cell's machinery.
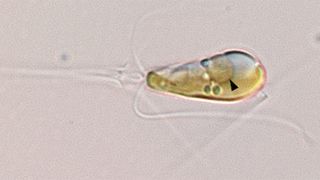
In a groundbreaking discovery, scientists uncovered the first known structure in complex cells that's capable of drawing nitrogen from the atmosphere and converting it into a form that the cell can use.
They've dubbed the newfound cell part the "nitroplast." And according to two recent studies, the researchers think it likely evolved 100 million years ago.
The nitroplast probably developed from a bacterium in the ocean, after the microbe was engulfed by an algal cell. The bacteria and algae were previously thought to be living in symbiosis, with the microbe supplying nitrogen in a form the algae could use and the algae providing the microbe with a home.
But it turns out that the microbe took on a new form long ago, becoming a full-fledged cell structure, or organelle, with a metabolism directly linked to that of the algae.
Related: Does evolution ever go backward?
"It's very rare that organelles arise from these types of things," Tyler Coale , a postdoctoral scholar at the University of California, Santa Cruz (UCSC) and lead author of one of two recent studies that identified the nitroplast, said in a statement .
The discovery is only the fourth known example in Earth's history of "primary endosymbiosis," a process by which a eukaryotic cell — a cell where DNA is enclosed in a nucleus, as in all animals, plants and fungi — swallows a prokaryotic cell, which lacks a nucleus. In this case, a eukaryotic algal cell swallowed a prokaryotic bacterial cell.
Sign up for the Live Science daily newsletter now
Get the world’s most fascinating discoveries delivered straight to your inbox.
"The first time we think it happened, it gave rise to all complex life," Coale said, referring to the evolution of mitochondria , the cells' powerhouses, approximately 1.5 billion years ago. "Everything more complicated than a bacterial cell owes its existence to that event." That includes humans.
The second known instance of endosymbiosis took place roughly 1 billion years ago, giving rise to chloroplasts, which power photosynthesis , and triggering the evolution of plants. The third known event may have given rise to a lesser-known organelle known as the chromatophore, a pigment-filled structure in the skin of cephalopods, such as squid and octopuses, that allows them to change color .
Scientists first discovered the microbe-turned-nitroplast in 1998, although at the time, they didn't yet know the microbe was a true organelle.
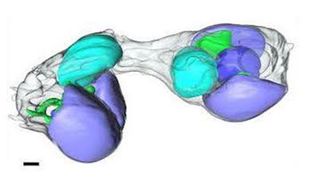
In that work, a team led by Jonathan Zehr , a distinguished professor of marine sciences at UCSC and lead author of the second recent study, recovered a short DNA sequence of the microbe from Pacific Ocean seawater. Zehr and his colleagues determined that the DNA belonged to a nitrogen-fixing cyanobacterium, which they called UCYN-A. (Nitrogen-fixing refers to the process of transforming nitrogen into a usable form for cells.)
The discovery coincided with work at Kochi University in Japan, where scientists figured out how to culture the algae that carry UCYN-A in the lab. This enabled Zehr and collaborators to compare the size of UCYN-A in different species of these algae, which belong to a related group called Braarudosphaera bigelowii.
The researchers published this work March 28 in the journal Cell , reporting that the growth of UCYN-A and its host cells are synchronized and controlled by the exchange of nutrients. This is "exactly what happens with organelles," Zehr said in the statement. "If you look at the mitochondria and the chloroplast, it's the same thing: they scale with the cell."
To confirm these results, Zehr and additional researchers conducted a second study, which was published April 11 in the journal Science . Its results indicated that UCYN-A imports proteins from its host cell, suggesting that the former microbe had ditched some of its cellular machinery, relying instead on its host to function. In other words, the once-bacterium had become a cog in the machinery of its host.
— Scientists stumble upon a new part of a cell in one of the most studied animals on Earth
— Meet the 'exclusome': A mini-organ just discovered in cells that defends the genome from attack
— Meet the 'frodosome,' a brand new organelle
"That's one of the hallmarks of something moving from an endosymbiont to an organelle," Zehr said. "They start throwing away pieces of DNA, and their genomes get smaller and smaller, and they start depending on the mother cell for those gene products — or the protein itself — to be transported into the cell."
UCYN-A also replicates at the same time as its host cell and is inherited like other organelles, sealing the discovery of the nitroplast, according to the statement.
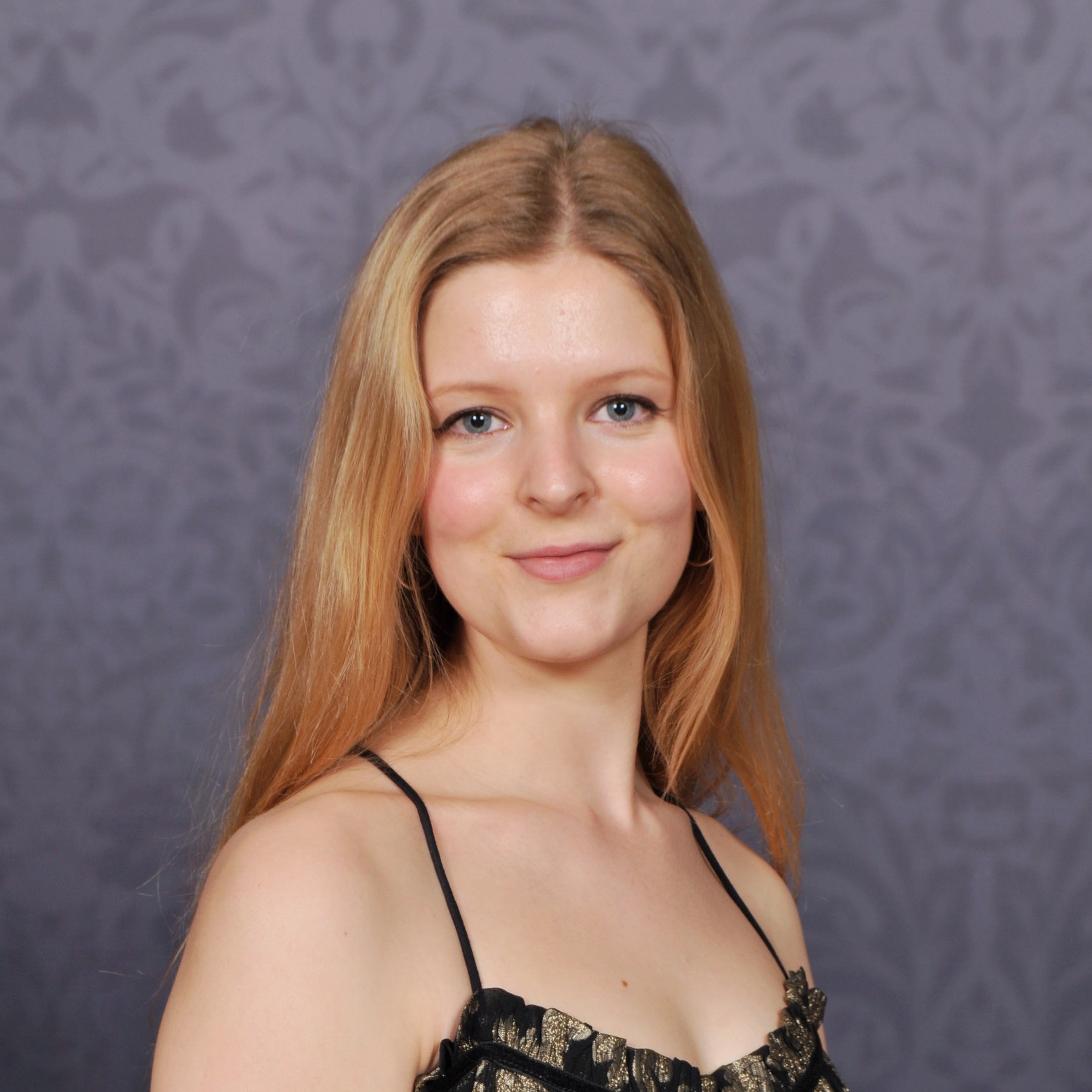
Sascha is a U.K.-based trainee staff writer at Live Science. She holds a bachelor’s degree in biology from the University of Southampton in England and a master’s degree in science communication from Imperial College London. Her work has appeared in The Guardian and the health website Zoe. Besides writing, she enjoys playing tennis, bread-making and browsing second-hand shops for hidden gems.
Hidden 'biosphere' of extreme microbes discovered 13 feet below Atacama Desert is deepest found there to date
Lost world of lagoons filled with mounds of microbes discovered in Atacama desert
Lasers reveal prehistoric Irish monuments that may have been 'pathways for the dead'
Most Popular
- 2 Giant, 82-foot lizard fish discovered on UK beach could be largest marine reptile ever found
- 3 Global 'time signals' subtly shifted as the total solar eclipse reshaped Earth's upper atmosphere, new data shows
- 4 Scientists discover once-in-a-billion-year event — 2 lifeforms merging to create a new cell part
- 5 NASA's downed Ingenuity helicopter has a 'last gift' for humanity — but we'll have to go to Mars to get it
- 2 Haunting photo of Earth and moon snapped by China's experimental lunar satellites
- 3 DNA analysis spanning 9 generations of people reveals marriage practices of mysterious warrior culture
- 4 5 catastrophic megathrust earthquakes led to the demise of the pre-Aztec city of Teotihuacan, new study suggests
- 5 'We were in disbelief': Antarctica is behaving in a way we've never seen before. Can it recover?

IMAGES
VIDEO
COMMENTS
1. Structure of the Interphase Nucleus. The nucleus is the largest organelle in the cell. A typical electron microscope image of a nucleus, the largest eukaryotic organelle in a cell, is shown below. This cross-section of an interphase nucleus reveals its double membrane, or nuclear envelope. The outer membrane of the nuclear envelope is ...
Take a short, narrated trip through a cell to see the nucleus, DNA, ribosomes, mitochondria, and more in this immersive Virtual Reality video!HOW TO: If you ...
8 years ago. The Endoplasmic Reticulum in a eukaryotic cell is the transport network of the cell and it extends from and connects the nuclear membrane to the plasma membrane of a cell. But then whenever we draw a diagram of a typical plant or animal cell, we never extend it to the plasma membrane- we always leave it somewhere in the cytoplasm.
Paul Andersen takes you on a tour of the cell. He starts by explaining the difference between prokaryotic and eukaryotic cells. He also explains why cells ...
Introduction to eukaryotic cells. By definition, eukaryotic cells are cells that contain a membrane-bound nucleus, a structural feature that is not present in bacterial or archaeal cells. In addition to the nucleus, eukaryotic cells are characterized by numerous membrane-bound organelles such as the endoplasmic reticulum, Golgi apparatus, chloroplasts, mitochondria, and others.
Tour of a eukaryotic cell. Join us on a voyage through some of the specialized compartments and structures of eukaryotic cells. From the rolling folds of the endoplasmic reticulum to the majestic fibers of the microtubule cytoskeleton, it's a trip you won't regret! Learn.
Eukaryotic cell structures. Google Classroom. The diagram below represents a cell found in eukaryotic organism. The magnified section represents an organelle found in this cell, labeled X. What is the function of organelle X? Choose 1 answer: (Choice A) Organelle X is where genetic information is stored.
1.4.1. The Nucleus. The nucleus is the largest organelle in the cell, separating the genetic blueprint (DNA) from the cell cytoplasm. Although the eukaryotic nucleus breaks down during mitosis and meiosis as chromosomes form and cells divide, it spends most of its time in its familiar form during interphase, the time between cell divisions.The structural organization of an interphase nucleus ...
In this episode of Crash Course Biology, we'll take a look at the difference between prokaryotic and eukaryotic cells, take a guided tour of the eukaryotic cell, and learn why most cells are small. We'll explore the eukaryotic cell's surprising beginnings through an endosymbiosis that occurred about 1.5 billion years ago. Transcript.
Compares and contrasts prokaryote cells and eukaryote cells before exploring organelle structures and functions! Video includes the modern cell theory and p...
1 Introducing the cell. Studies into the function and appearance of cells reveal the presence of great diversity, which is particularly striking among eukaryotic cells. Even very different types of eukaryotic cells, however, exhibit many common structures and functions. This mix of uniformity and diversity is also reflected in the organisation ...
Eukaryotic cells also have organelles, which are membrane-bound structures found within the cell. If you looked at eukaryotic cells under a microscope, you'd see distinct structures of all shapes and sizes. Prokaryotic cells, on the other hand, would look more uniform because they don't have those membrane-bound structures to break up the cell.
Lesson 3: Tour of a eukaryotic cell. Endoplasmic reticulum and Golgi bodies. Endomembrane system. The endomembrane system. Mitochondria. Mitochondria and chloroplasts. The cytoskeleton. ... It was originally thought that the cytoskeleton was unique to eukaryotic cells, but many proteins analagous to those found in eukaryotic cells have now been ...
The eukaryotic cells types are generally found in animals, plants, algae, and fungi. For the purpose of this article, the primary focus will be the structure and histology of the animal cell. The major differences between animal and plant cells will be explored as well. As previously stated, the fundamental components of a cell are its organelles.
Reveal answer. Below, a 'tour' through the eukaryotic cell describes the different subcellular components and their functions, with an emphasis on a typical higher (mammalian) animal cell, but also describing some of the cell components in other eukaryotic organisms. It should become clear, as you work through this section, that eukaryotic ...
The question of how life began has been with us since the beginnings or recorded history. It is now accepted that there was a time, however brief or long, when the earth was a lifeless (prebiotic) planet. Life's origins on earth date to some 3.7-4.1 billion years ago under conditions that favored the formation of the first cell, the first ...
Eukaryotic cells are generally much bigger than prokaryotic cells. The logistics of carrying out metabolism set limits on cell size. At the lower limit, the smallest bacteria, mycoplasmas, are between 0.1 to 1.0 micron. Most bacteria are 1-10 microns in diameter. Eukaryotic cells are typically 10-100 microns in diameter.
Most of the discussion of cell structure that follows in this chapter applies to eukaryotic cells. A Panoramic View of the Eukaryotic Cell Figure 6.8 - Two eukaryotic cells: 1) animal cell, and 2) plant cell. Note the similarities and differences between each. Concept 6.3: The eukaryotic cell's genetic instructions are housed in the nucleus ...
Mitochondria. Mitochondria are organelles that contain their own DNA, and have both inner and outer membranes. Mitochondria are often referred to as the "powerhouses of the cell" because they are responsible for producing most of the cell's energy in the form of ATP. According to the endosymbiont theory, mitochondria began as separate bacteria ...
Actin tracks use myosin motors to move materials short distances inside the cell. Stress fibers bear tension, resist pulling forces. The cortex just inside the plasma membrane supports the cell's shape. Actin bundles make up the core of microvilli. Actin treadmills form the basis for cell migration.
A Tour of the Cell. Paul Andersen takes you on a tour of the cell. He starts by explaining the difference between prokaryotic and eukaryotic cells. He also explains why cells are small but not infinitely small. He also explains how the organelles work together in a similar fashion.
The eukaryotic cell is a lot like that large space, with lots of interior rooms (i.e., organelles) with their own entryways and exits. In fact, eukaryotic life would not even be possible without a division of labor of eukaryotic cells among different organelles (the equivalence to the small rooms in our metaphor).
Eukaryotic cells, apart from the cytoskeleton, are distinctive than Prokaryotic cells for having membrane-bound organelles. Organelles speak of a higher level of organization. That requires more space, because there are more and more organelles which need to be able to fit into one cell while not being squashed tightly into each other.
The discovery is only the fourth known example in Earth's history of "primary endosymbiosis," a process by which a eukaryotic cell — a cell where DNA is enclosed in a nucleus, as in all animals ...