Create an account
Create a free IEA account to download our reports or subcribe to a paid service.
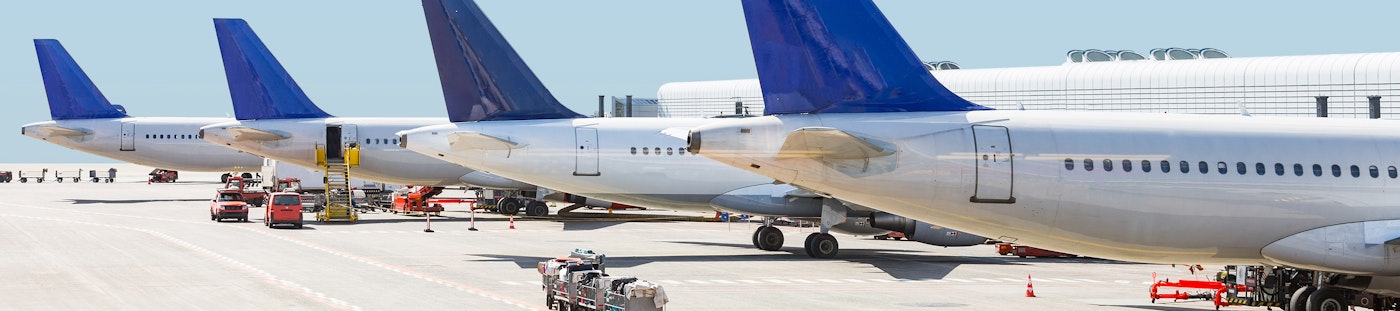

Why is aviation important?
Aviation accounts for a relatively small share of global emissions but is one of the most challenging sectors to decarbonise. Despite reductions in flying during the Covid-19 lockdowns, demand is expected to grow rapidly through 2030. New aircraft can be up to 20% more efficient than the models they replace, but growth in activity has historically outpaced efficiency improvement.
Where do we need to go?
Technology innovation is needed across the sector, including in production of low-emission fuels, improvements in aircraft and engines, and operational optimisation. Demand restraint solutions will also be needed to get on track with the Net Zero Emissions by 2050 Scenario – to curb growth in emissions and ultimately reduce them this decade.
What are the challenges?
Planned production capacity for sustainable aviation fuels will provide just a small fraction of jet fuel demand by 2027. Increasing the use of these fuels to get in line with the Net Zero Scenario will require supportive policies and a significant ramp-up of investments in production capacity.
Why is aviation important? Chevron down
Where do we need to go chevron down, what are the challenges chevron down, tracking aviation.
In 2022 aviation accounted for 2% of global energy-related CO 2 emissions, having grown faster in recent decades than rail, road or shipping. As international travel demand recovers following the Covid-19 pandemic, aviation emissions in 2022 reached almost 800 Mt CO 2 , about 80% of the pre-pandemic level. Many technical measures related to low-emission fuels, improvements in airframes and engines, operational optimisation and demand restraint solutions are needed to curb growth in emissions and ultimately reduce them this decade in order to get on track with the Net Zero Emissions by 2050 (NZE) Scenario.
Country and regional highlights Chevron down
Political agreement on targeting net zero for aviation has been complemented by fiscal and regulatory policies to promote sustainable aviation fuels in major markets
Notable progress towards getting aviation on track includes the following:
- The 184 member states of the International Civil Aviation Organization (ICAO) adopted in 2022 a long-term global aspirational goal (LTAG) of net zero carbon emissions from international aviation by 2050.
- In 2022 the United States announced important tax credits and a competitive grant programme under the Inflation Reduction Act , which will allocate USD 3.3 billion to scaling up sustainable aviation fuel (SAF) production, with the aim of meeting its 3 billion gallons milestone by 2030 .
- In the European Union, the European Parliament and Council reached a political agreement in 2023 on the rules of ReFuelEU Aviation on the schedule of minimum SAF blend-in shares, with sub-targets for synthetic fuels, through 2050.
- In 2022, following its announced Jet Zero pledge, the United Kingdom dedicated GBP 165 million to support SAF projects, with a plan to have at least 5 commercial SAF plants under construction by 2025.
CO2 emissions Chevron down
CO2 emissions from aviation have reached 80% of their pre-pandemic peak
CO2 emissions in aviation in the Net Zero Scenario, 2000-2030
Aviation emissions rose in 2022 to reach nearly 80% of their pre-pandemic peak in 2019. After increasing at an average of 2.3% per year from 1990 to 2019, direct CO 2 emissions from fossil fuel combustion plummeted from more than 1 000 Mt CO 2 in 2019 to less than 600 Mt CO 2 in 2020 in the context of the Covid-19 pandemic. As demand from air passengers recovered in 2022, emissions increased in all regions with the exception of China (due to the zero-Covid policy) and Russia (due to the invasion of Ukraine), reaching almost 800 Mt CO 2 . CO 2 emissions are expected to grow rapidly and to surpass their 2019 level around 2025.
Multiple measures are required to promote the technologies, sustainable aviation fuels (SAF) and demand-side management needed to bring the currently rising emissions level below 1 000 Mt CO 2 by 2030, in line with the NZE Scenario. Policy and fiscal support should prioritise improvements in energy efficiency, stimulating investment in pre-commercial and low-emissions SAF, and developing alternatives to jet kerosene, such as electricity- or hydrogen-powered aircraft.
Energy Chevron down
Improvements in energy intensity have not been sufficient to counterbalance energy demand growth in recent years
Energy intensity of commercial passenger aviation in the Net Zero Scenario, 2000-2030
From 2010 to 2019, average fuel efficiency per revenue tonne kilometre (RTK) equivalent travelled improved by 1.8% per year thanks to the introduction of more efficient aircraft and engines , with improvements over the decade nearly reaching ICAO’s aspirational goal of 2% per annum through 2050. However, efficiency improvements have not kept up with demand growth, which grew at an average rate of over 5% annually between 2010 and 2019. To stay on track with the Net Zero Scenario, efficiency will need to improve at a rate of 2% per year through 2030, on a par with the historical average improvements over the past two decades.
In addition to technical efficiency improvements in engine and airframe designs, improvements in payload and traffic efficiency (i.e. the weight of cargo and number of passengers carried per aircraft) have also contributed to reducing the energy intensity of aircraft operation. Payload efficiency deteriorated between 2020 and 2022, as planes were flown with fewer passengers despite some compensatory increase in freight payloads in the bellies of passenger aircraft.
Activity Chevron down
International passenger activity more than doubled in 2022 compared to the previous year, closing the gap with pre-Covid levels
Global total commercial air passenger traffic, 2019-2022
Total commercial (international plus domestic) air passenger activity increased by around 70% in 2022, and now stands at three-quarters of pre-pandemic levels. Increasing activity in international aviation was the main driver, with more than 150% growth , after a year of slower recovery compared to domestic aviation. The reopening of international travel in China provides an outlook for even higher growth from the beginning of 2023, as passenger and cargo activity return to pre-pandemic levels.
Air cargo contracted by 8% in 2022 after a historical peak in 2021, as a result of high inflation, disruption of trade flows due to the war in Ukraine, and the strong US dollar making commodities traded in US currency more expensive.
Technology deployment Chevron down
Sustainable aviation fuels are critical to decarbonising aviation
Currently, demand for aviation fuel is dominated by jet kerosene, while SAF account for less than 0.1% of all aviation fuels consumed. Manufacturers and operators are increasingly testing flights that are entirely fuelled by SAF, which can be deployed in current infrastructure, engines and aircraft with minor adjustments to fuel delivery equipment. However, planned production capacities will provide just 1-2% of jet fuel demand by 2027. Increasing SAF use in aviation to 10% by 2030 in line with the Net Zero Scenario will require a significant ramp-up of investment in capacity to produce SAF and supportive policies such as fuel taxes and low-carbon fuels standards.
Commercial adoption of SAF will be driven by policies such as the aforementioned incentives for SAF production in the United States and the United Kingdom , and the European Union’s proposed ReFuelEU regulation. The current ReFuelEU Aviation proposal excludes crop-based SAF due to sustainability concerns, and the potential to scale up biojet kerosene supply based on animal fat and waste oil feedstocks (which are included in the proposed regulation) is limited by their supply. Supporting research and development on advanced biofuel technologies can unlock more abundant and cheaper feedstocks such as agricultural residues, dedicated energy crops and municipal solid waste. In the longer term, synthetic fuels based on hydrogen produced using electrolysers (and running on low-emissions or renewable electricity), combined with CO 2 from biogenic, concentrated waste streams or atmospheric sources can provide an alternative, although commercialisation may be challenging.
Revolutionary designs are needed to achieve efficiency improvements of more than 2% annually in the longer term
Incremental improvements to engines, materials, aerodynamics and mild hybridisation can and should be implemented. However, “revolutionary” designs , such as new airframe configurations to enable further efficiency improvement, and alternative propulsion technologies including electric or hydrogen-powered aircraft, may also play a role.
Innovation Chevron down
Tests and prototypes showcase innovations in hydrogen-powered aircraft
Hydrogen can be used via direct combustion in jet engines, or in fuel cells to generate electricity for electric motors, or a combination of the two. However, using hydrogen in aircraft poses significant challenges, including the need for innovative fuel storage and delivery methods, low-cost and lightweight cryogenic tanks, and redesigned airframes to accommodate them. By 2040 hydrogen aircraft are likely to have a maximum range of under 3 500 km , serving at a maximum about half of all fuel consumption in current commercial aviation operations.
Test flights for aircraft equipped with fuel cell-powered electric motors have taken place in early 2023, with ZeroAvia flying a 19-seat aircraft equipped with a hydrogen-electric engine on its left wing, and Universal Hydrogen flying a 40-seat regional aircraft at high altitude, with one of its engines powered by fuel cell. This followed its demonstration of prototype modular capsules that can store liquid hydrogen for up to 100 hours and can be transported by semi-truck.
Demonstrations of alternative propulsion systems have also progressed from late 2022 onwards. Airbus 's ZEROe programme produced a prototype of a cryogenic hydrogen tank that allows hydrogen to be carried in its liquid form at extremely low temperatures. Rolls-Royce and easyJet tested combusting hydrogen to run a regional jet engine with hydrogen produced from wind and tidal power. Avio Aero has launched a demonstration programme for megawatt-level hybrid electric propulsion technologies, coupling a propulsion engine with a fuel cell-powered electric motor. H2FLY has begun the integration of a liquid hydrogen storage system tank in its four-seat aircraft with hydrogen-electric propulsion.
Battery electric propulsion is currently limited to very small aircraft and short range
Battery electric aircraft have no direct emissions, potentially much lower operational and maintenance costs (dependent on battery durability), high efficiency and create far lower noise pollution. However, current battery energy density and weight severely restrict the range of battery electric flights and the size of the aircraft. In September 2022 Eviation performed a test flight of its 9-seat electric aircraft, with maximum flight range of around 450 km.
The success of deploying electric aircraft technologies will largely depend on the evolution of battery technologies. The energy density of today’s Li-ion batteries is around 200 Wh/kg (with a current practical limit of 300-400 Wh/kg) at the pack level, but for short-haul flights over 1 000 km, a battery pack energy density of at least 800 Wh/kg would be needed.
World’s first airport liquid hydrogen refuelling facility under construction
Airbus and ArianeGroup are working towards building the first liquid hydrogen refuelling facility for ZEROe aircraft at Blagnac airport in Toulouse, France.
Policy Chevron down
Governments are increasing fiscal support for SAF production and mandating SAF use
Production of SAF gained substantial support through the passage in 2022 of the Inflation Reduction Act (IRA) in the United States, with USD 3.3 billion in tax credits and a competitive grant programme allocated to SAF. The IRA provides USD 1.25 per gallon (USD 0.33 per litre) of SAF produced, provided that the lifecycle emissions are no more than 50% of those of fossil jet kerosene. A supplemental 1 cent credit is available for each percent that the lifecycle emissions reduction exceeds 50%.
In addition to amending the European Union Emissions Trading System to phase out allowances given to the aviation industry by 2026, in Q1 2023 the European Council and Parliament agreed on rules mandating a minimum share of SAF with sub-targets for synthetic fuels. Individual countries such as France and Norway already have SAF blending mandates in place, while Sweden’s GHG emissions intensity reduction target will also drive SAF adoption. In 2023 the Swedish government also announced its aim to invest SEK 15 million (Swedish kronor) annually to support research and development of electric aircraft. The United Kingdom, following its 2022 Jet Zero pledge, has dedicated GBP 165 million to support SAF projects, with funding allocation running to 2025.
In the Asia Pacific region, in 2022 Japan proposed legislation mandating that SAF must account for 10% of aviation fuel by 2030. In the same period the Civil Aviation Administration of China also set ambitions to increase SAF use and lower GHG emissions intensity.
View all aviation policies
- Policies and Measures database (PAMS) circle-arrow
International collaboration Chevron down
International aviation has pledged to reach net zero emissions by 2050
In late 2022, ICAO member states adopted a long-term global aspirational goal (LTAG) to achieve net zero carbon emissions from international aviation by 2050. The agreement aims to reduce emissions within the sector itself (i.e. directly from aviation activity, as opposed to via offsetting emissions through purchase of credits). Although it remains non-binding and lacks intermediate goals, member state governments are expected to produce action plans within their own national timeframe and capabilities.
Additionally, countries agreed on a new baseline for the Carbon Offsetting and Reduction Scheme for International Aviation (CORSIA) at 85% of the 2019 emissions level of international aviation. Emissions beyond this would need to be reduced by using sustainable aviation fuels or by purchasing certified emissions reductions to offset CO 2 emissions. ICAO approved addition programmes for CORSIA compliance during the pilot phase, many of which rely on offsets outside the energy sector.
Private sector strategies Chevron down
Airlines are moving towards offtake agreements with fuel suppliers to supply SAF
Increasing announcements of SAF offtake agreements between fuel suppliers and airlines marked a stark increase in contracted volume from 9 billion litres in 2021 to 22 billion litres in 2022. The largest offtake volume per year purchase to date was agreed between Gevo and OneWorld Alliance , to be sourced from ethanol from inedible corn products. Many of the contracted volumes have planned delivery after 2025, as new SAF plants take around three years to build after a final investment decision has been taken.
Acknowledgements Chevron down
We would like to thank the following external reviewers:
- Lynnette Dray, University College London
- Haldane Dodd, Air Transport Action Group
Recommendations
1 supply-side and demand-side policies will need to mutually reinforce each other to scale up saf production and demand chevron down.
Policies to support SAF consumption and boost growth are needed to make SAF more widely available and economically viable.
On the demand side, blending mandates provide clear long-term demand signals beyond offtake agreements. Low-carbon fuel standards provide less clarity on required volumes, but this is balanced by providing a clear regulatory framework to reduce the lifecycle emissions of SAF. By combining blending mandates with GHG emissions and sustainability criteria, regulatory frameworks such as the proposed ReFuelEU Aviation aim to achieve market certainty at the same time as incentivising uptake of less mature and higher cost SAF production pathways in the mid- to long term.
Funding and financial de-risking will be needed to promote continued innovation around sustainable production processes including novel feedstocks (wastes, residues, marginal land, double cropping) and support the leap from demonstration to commercial plants. This will also be needed to drive investment at all stages of research, and to enable power-to-liquids jet kerosene to scale up rapidly.
Both supply and demand of SAF are highly concentrated in advanced economies today. Meeting the growing demand for air travel in emerging and developing economies will require technical assistance and capacity building to accelerate the availability and use of SAF. Such support can help emerging market and developing economies to leverage domestic natural resource endowments such as biomass, solar and wind, and thereby scale up high value-add industries in the clean energy economy.
2 Tax aviation fuels according to impact, acknowledging that only a minority of the world flies Chevron down
Taxing GHG emissions beyond the CORSIA scheme is critical to more equitably reflect the climate impacts of air travel. As the additional costs of these taxes are passed on to passengers, they can help curb demand growth, while revenues generated could be used to foster low-emission innovation in SAF production or engine and airframe design.
Since frequent fliers likely account for the majority of commercial aviation emissions , progressive tax rates that increase with flight frequency as well as higher taxes on premium class tickets could discourage excessive flying, especially as jet kerosene is currently taxed at lower levels than residential electricity or automative fuels in many regions. However, changing tax structure is complicated by taxation conditions on international flights agreed in the Chicago Convention of 1944 .
3 The aviation sector can lead on bringing nascent decarbonisation options to scale Chevron down
Certain airlines propose offset options to consumers, such as paying extra towards SAF . Some have set corporate targets to supply increasing shares of fuels with SAF , and are actively seeking offtake agreements that secure demand for nascent SAF markets.
Actions from leading airlines and airports that serve as key international and domestic hubs can generate the market pull that is needed to catalyse adoption of efficient operations, best-in-class technologies and SAF. Those that act early will benefit not only from asserting their leadership in corporate social responsibility, but from being the first to gain experience of innovative practices and technologies that will eventually need to be adopted more widely. Investors, lenders and consumers will be instrumental in driving uptake of SAF by funding airlines and setting corporate travel and freight transport policies.
Last update on 11 July 2023
Programmes and partnerships
Technology collaboration programme.
Advancing the research, development and commercialisation of energy technologies
Renewables 2022
Biojet fuel demand expands to 3 900 MLPY in our main-case forecast – 37 times the 2021 level – to account for nearly 1% of total jet fuel consumption.
Fuel report — December 2022
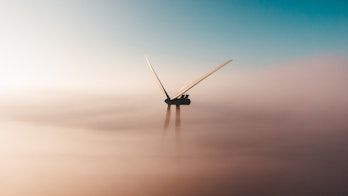
Authors and contributors
Lead authors Hyeji Kim Jacob Teter
Subscription successful
Thank you for subscribing. You can unsubscribe at any time by clicking the link at the bottom of any IEA newsletter.
- Share full article
Advertisement
Supported by
Nations Agree to Curb Emissions From Flying by 2050
The agreement, years in the making, is a milestone in the effort to reduce greenhouse gas emissions from airplanes worldwide.

By Hiroko Tabuchi
After almost a decade of talks, the nations of the world committed Friday to drastically lower emissions of planet-warming gases from the world’s airplanes by 2050, a milestone in efforts to ease the climate effects of a fast-growing sector.
The target to reach “net zero” emissions — a point in which air travel is no longer pumping any additional carbon dioxide into the atmosphere — would require the aviation industry to significantly step up its climate efforts. Previously, companies had relied on offsetting aviation’s emissions growth through tree-planting programs or through yet-to-be-proven technology to pull carbon dioxide out of the air.
But to reach net zero, companies and governments would need to invest hundreds of billions of dollars in increasingly efficient planes and cleaner fuels to sharply reduce emissions from air travel itself. And even those investments are unlikely to be enough, compelling countries and companies to adopt policies to curb flying itself, by scrapping fuel subsidies or halting airport expansion plans, for example, or ending frequent flier programs.
That puts the onus on the world’s richest countries, which account for the bulk of global air travel. The richest 20 percent of people worldwide take 80 percent of the flights, according to estimates by the International Council on Clean Transportation, a nonprofit think tank. The top 2 percent of frequent fliers take about 40 percent of the flights.
“To build room for poorer countries to grow their aviation sectors, richer countries will need to peak emissions even faster,” said Dan Rutherford, director of the think tank’s aviation and marine programs.
Emissions from global commercial aviation made up about 3 percent of global emissions in 2019, and had surged more than 30 percent over the previous decade before the coronavirus pandemic hit and traffic slumped. But air travel has come back with a vengeance, making action to address rising emissions imperative.
The aviation industry has been slow to address its emissions, which aren’t covered by the Paris accord, the 2015 agreement among the nations of the world to fight climate change. Instead, a United Nations-like body called the International Civil Aviation Organization has overseen the climate talks. Those talks quickly became a microcosm of the politics involved in global climate negotiations, with less wealthy nations arguing that they should not face the same restrictions as richer nations.
India and China, where air travel is surging, argued at the talks in Montreal this week that their air carriers would require until 2060 or 2070 to achieve net zero emissions.
The 2050 target comes with no guarantees of success. Similar to the Paris agreement, ICAO’s targets don’t come with any authority to set policy. In addition, the agreement doesn’t assign targets to specific countries or airline companies, leaving the task of setting rules to member states.
Aviation’s contribution to climate change has been thrust into the spotlight in recent years by climate activists like Greta Thunberg , the Swedish environmentalist who inspired a worldwide no-fly campaign.
The European Union has also taken the lead in policy, proposing a plethora of changes like ending tax exemptions for jet fuel and taxing carbon emissions instead. French politicians have also proposed banning short-haul flights. In the United States, the ambitious Inflation Reduction Act passed this year includes subsidies for sustainable aviation fuel.
Hiroko Tabuchi is an investigative reporter on the Climate desk. She was part of the Times team that received the 2013 Pulitzer Prize for explanatory reporting. More about Hiroko Tabuchi
Learn More About Climate Change
Have questions about climate change? Our F.A.Q. will tackle your climate questions, big and small .
Paris is becoming a city of bikes. Across China, people are snapping up $5,000 electric cars. Here’s a look at a few bright spots for emission reductions.
In theory, online shopping can be more efficient than driving to the store. But you may still want to think before you add to cart.
“Buying Time,” a new series from The New York Times, looks at the risky ways humans are starting to manipulate nature to fight climate change.
Big brands like Procter & Gamble and Nestlé say a new generation of recycling plants will help them meet environmental goals, but the technology is struggling to deliver .
Did you know the ♻ symbol doesn’t mean something is actually recyclable ? Read on about how we got here, and what can be done.
Aviation is responsible for 3.5 percent of climate change, study finds
- September 3, 2020
New research that provides the most comprehensive calculations of aviation’s impact on the climate finds that global air travel and transport is responsible for 3.5 percent of all drivers of climate change from human activities.
The study, published in the journal Atmospheric Environmen t , evaluated all of the aviation industry’s contributing factors to climate change, including emissions of carbon dioxide (CO 2 ) and nitrogen oxide (NOx), and the effect of contrails and contrail cirrus – short-lived clouds created in jet engine exhaust plumes at aircraft cruise altitudes that reflect sunlight during the day and trap heat trying to escape at night.
The findings show that two-thirds of the impact from aviation is attributed to contrails, NOx, water vapor, sulfate aerosol gases, soot, and other aerosols. The remainder is due to the cumulative heat-trapping effects of long-lived CO 2 emissions – 32.6 billion tonnes between 1940 and 2018, or roughly the total global CO 2 emissions for the year 2010.
The five-year research effort was led by the UK’s Manchester Metropolitan University in collaboration with numerous academic and research institutions across the globe.
Co-author David Fahey, director of NOAA’s Earth System Research Laboratories in Boulder, Colorado, said the research strengthens the scientific foundation for understanding the role of aviation in climate change.
“Our assessment will aid decision makers and the industry in pursuing any future mitigation actions, while protecting this important sector from any inaccurate assertions concerning its role in the climate system,” Fahey said.
The study presents the complete first set of calculations for aviation based on a new metric introduced in 2013 by the Intergovernmental Panel on Climate Change called ‘effective radiative forcing,’ or ERF. ERF represents the increase or decrease in the balance between the energy coming from the sun and the energy emitted from the Earth since pre-industrial times.
Using the new ERF metric, the team found that while contrail cirrus has the largest climate warming impact, it is less than half that previously estimated. The effects of CO 2 emissions generated by aviation last for many centuries, and represent the second largest contribution. Approximately half the total cumulative emissions of CO 2 were generated in the past 20 years.
“Given the dependence of aviation on burning fossil fuel, its significant CO 2 and non-CO 2 effects, and the projected fleet growth, it is vital to understand the scale of aviation’s impact on present-day climate change,” said lead author David Lee, professor of Atmospheric Science at Manchester Metropolitan University and Director of its Centre for Aviation, Transport, and the Environment research group.
Lee said that estimating aviation’s non-CO 2 effects on atmospheric chemistry and clouds was a complex challenge. “We had to account for contributions caused by a range of atmospheric physical processes, including how air moves, chemical transformations, microphysics, radiation, and transport.”
The new study will allow aviation’s impact on climate change to be compared with other sectors such as maritime shipping, ground transportation and energy generation as there is now a consistent set of estimates.
This story was adapted from a press release issued by Manchester Metropolitan University .
For more information, contact Theo Stein, NOAA Communications, at [email protected] .

No sign of greenhouse gases increases slowing in 2023

First-of-its-kind experiment illuminates wildfires in unprecedented detail
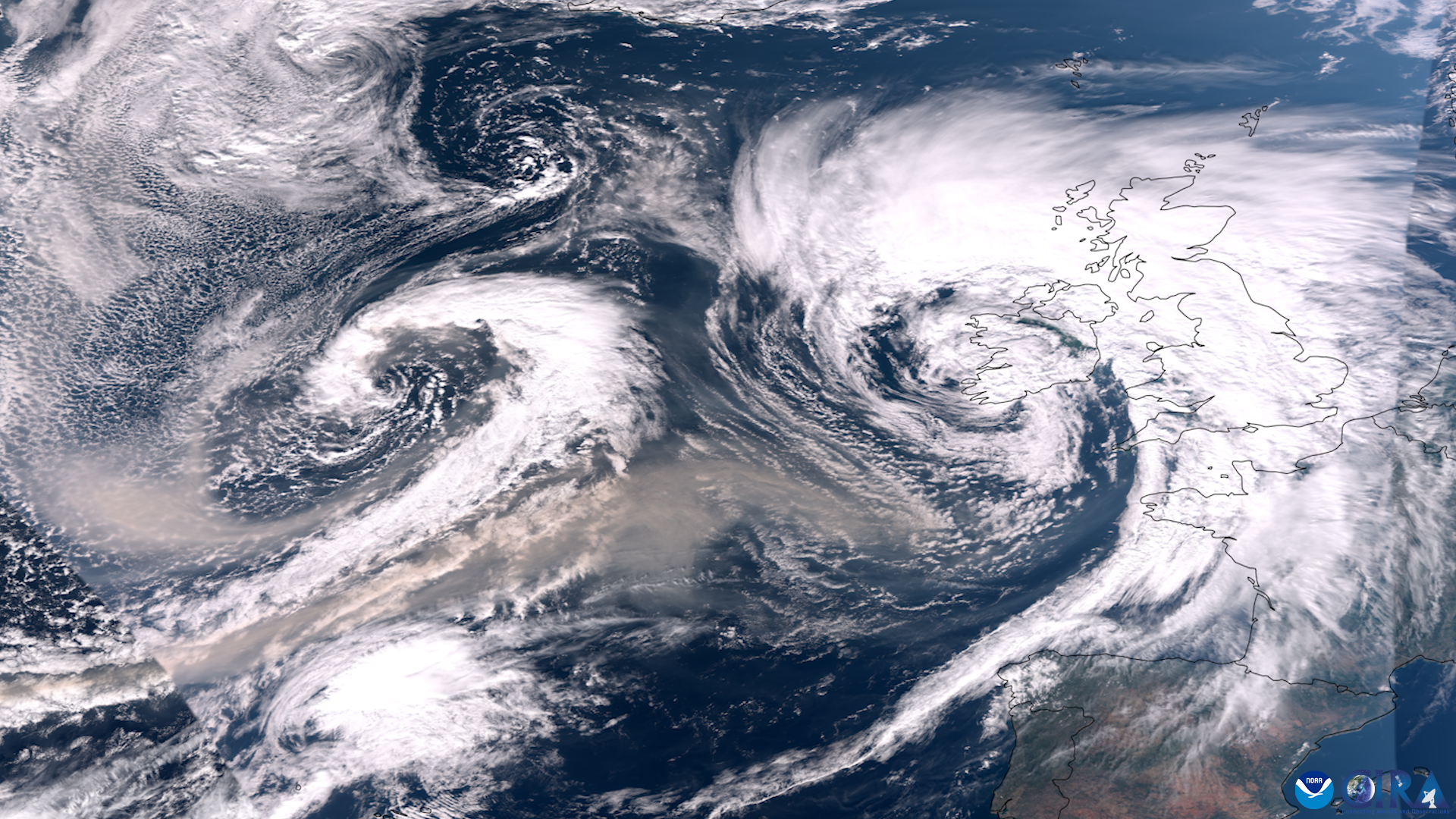
5 science wins from the 2023 NOAA Science Report
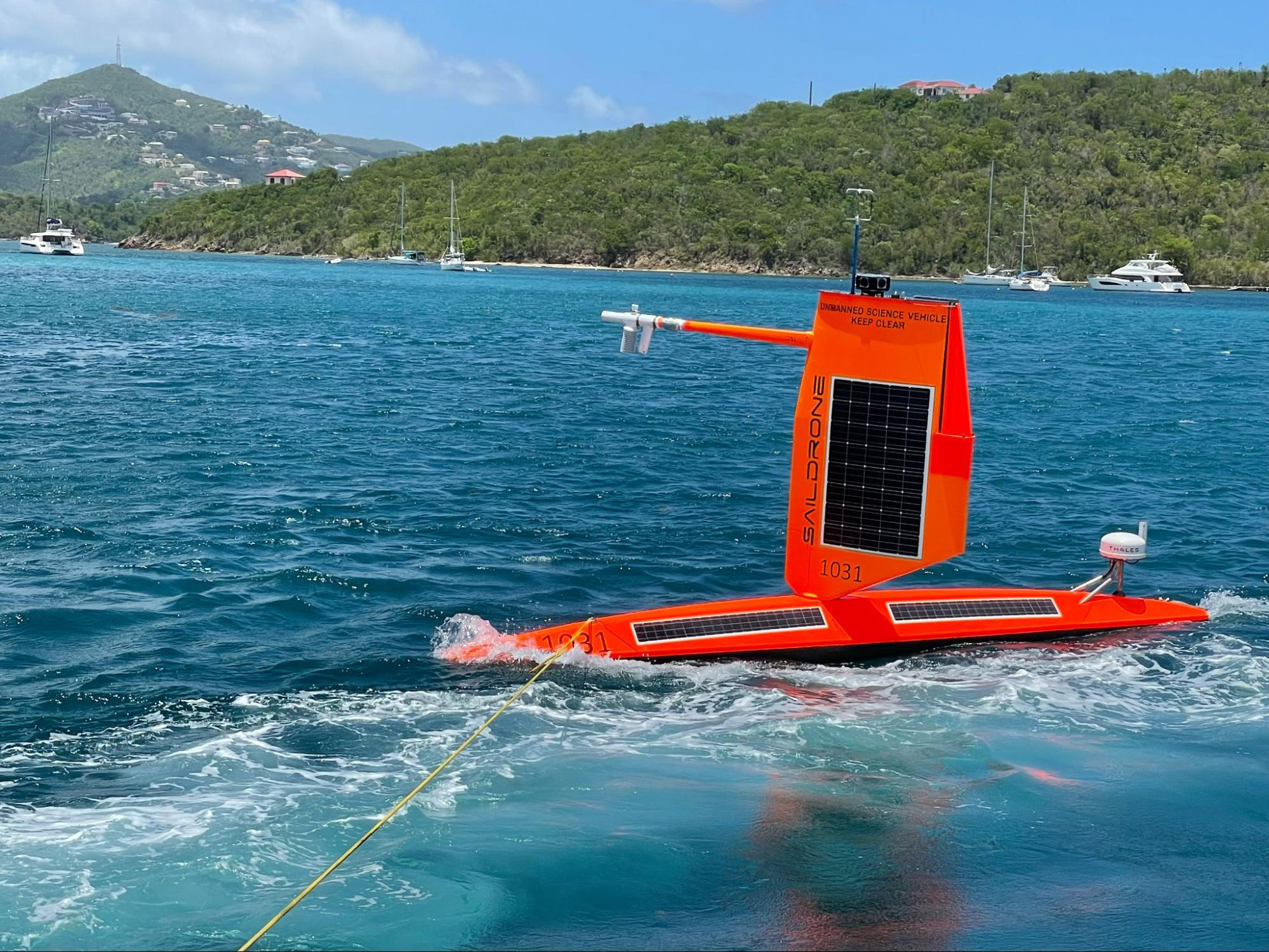
Filling A Data Gap In The Tropical Pacific To Reveal Daily Air-Sea Interactions
Popup call to action.
A prompt with more information on your call to action.
Thank you for visiting nature.com. You are using a browser version with limited support for CSS. To obtain the best experience, we recommend you use a more up to date browser (or turn off compatibility mode in Internet Explorer). In the meantime, to ensure continued support, we are displaying the site without styles and JavaScript.
- View all journals
- Explore content
- About the journal
- Publish with us
- Sign up for alerts
- Published: 30 January 2023
Pathways to net-zero emissions from aviation
- Candelaria Bergero 1 ,
- Greer Gosnell 2 ,
- Dolf Gielen 3 ,
- Seungwoo Kang 3 ,
- Morgan Bazilian 4 &
- Steven J. Davis ORCID: orcid.org/0000-0002-9338-0844 1 , 5
Nature Sustainability volume 6 , pages 404–414 ( 2023 ) Cite this article
32k Accesses
35 Citations
669 Altmetric
Metrics details
- Climate-change mitigation
- Climate-change policy
- Energy supply and demand
International climate goals imply reaching net-zero global carbon dioxide (CO 2 ) emissions by roughly mid-century (and net-zero greenhouse gas emissions by the end of the century). Among the most difficult emissions to avoid will be those from aviation given the industry’s need for energy-dense liquid fuels that lack commercially competitive substitutes and the difficult-to-abate non-CO 2 radiative forcing. Here we systematically assess pathways to net-zero emissions aviation. We find that ambitious reductions in demand for air transport and improvements in the energy efficiency of aircraft might avoid up to 61% (2.8 GtCO 2 equivalent (GtCO 2 eq)) and 27% (1.2 GtCO 2 eq), respectively, of projected business-as-usual aviation emissions in 2050. However, further reductions will depend on replacing fossil jet fuel with large quantities of net-zero emissions biofuels or synthetic fuels (that is, 2.5–19.8 EJ of sustainable aviation fuels)—which may be substantially more expensive. Moreover, up to 3.4 GtCO 2 eq may need to be removed from the atmosphere to compensate for non-CO 2 forcing for the sector to achieve net-zero radiative forcing. Our results may inform investments and priorities for innovation by highlighting plausible pathways to net-zero emissions aviation, including the relative potential and trade-offs of changes in behaviour, technology, energy sources and carbon equivalent removals.
Similar content being viewed by others
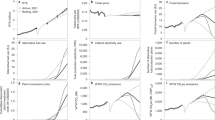
Cost and emissions pathways towards net-zero climate impacts in aviation
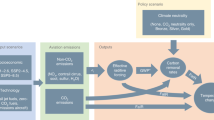
Definitions and implications of climate-neutral aviation
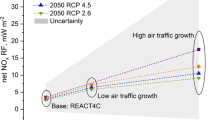
Greater fuel efficiency is potentially preferable to reducing NOx emissions for aviation’s climate impacts
Stabilizing global mean temperature at 1.5 °C above pre-industrial times means reaching net-zero CO 2 emissions (that is, balancing any ongoing emissions with removals) by 2050–2060, and net-zero greenhouse gas emissions by 2070–2100 1 . Large—and increasingly affordable—emissions reductions are available by improving energy efficiency, electrifying energy end uses and switching to non-emitting sources of electricity 1 , and many countries, subnational jurisdictions and companies have announced net-zero emissions targets 2 . However, flying will be particularly challenging to decarbonize because aircraft rely on energy-dense liquid hydrocarbons and flights also entail non-CO 2 radiative forcing 3 , 4 .
The climate impacts of global aviation are substantial, with one-third of radiative forcing related to CO 2 and two-thirds related mainly to nitrous oxides (NO x ) and water vapour in the form of contrail cirrus clouds 5 , 6 . According to the IEA, in 2019, aviation accounted for 1.03 GtCO 2 , or 3.1% of total global CO 2 emissions from fossil fuel combustion 7 , and 1.7 GtCO 2 equivalent (eq) when non-CO 2 forcing is included (based on a global warming potential of 100 years, or GWP100). Although emissions from air travel dropped 40% in 2020 due to the COVID-19 pandemic, aviation demand is expected to recover and grow in the future 8 , with emissions projected to reach as high as 1.9 GtCO 2 in 2050 9 ( ∼ 2.6 times 2021 values) or 3.4 GtCO 2 eq (GWP100). Demand for air travel across countries and population groups is closely associated with affluence and lifestyle 10 (Supplementary Fig. 1 ), and flying has become a lightning rod for climate activists who criticize the hypocrisy of climate scientists and climate-concerned policymakers who fly 11 .
Many aircraft manufacturers and industry groups aim to meet rising demand while also reducing emissions by improving operational efficiencies, offsetting carbon emissions and switching to net-zero emissions fuels 12 , 13 , 14 . Domestic aviation emissions are included in countriesʼ nationally determined contributions under the Paris climate agreement, but international aviation emissions are not. Recently, governments such as the United States (2021 Aviation Climate Action Plan) 15 and the European Union (Aviation Safety Agency report) 16 have addressed the sector’s emissions. Though most aviation-related climate targets have not been met 17 , in 2016, under the International Civil Aviation Organization (ICAO), 192 countries signed the Carbon Offsetting and Reduction Scheme for International Aviation (CORSIA) to make post-2020 growth of international aviation carbon neutral, either by fuel switching or by offsetting emissions 14 . Most prominently, the International Air Transport Association (IATA) committed in 2021 that emissions from global aviation would be net-zero by 2050 18 .
Recent analyses have evaluated the technological potential of powering aircraft with sustainable aviation fuels (SAFs) 3 , 19 , 20 , hydrogen or electricity 18 and offsetting aviation emissions by removing equivalent quantities of CO 2 from the atmosphere 21 (Supplementary Fig. 2 ). SAFs include biofuels and synthetic fuels that are ‘drop-in’ replacements for jet fuel (that is, they would require little or no changes to existing aircraft and fueling infrastructure) that meet ICAO’s sustainability criteria 14 of a net greenhouse gas emissions reduction on a life-cycle basis of at least 10% compared to fossil jet fuel, respecting biodiversity and contributing to local social and economic development.
Here we assess nine possible pathways to achieve net-zero direct emissions from aviation, including changes and trade-offs in demand, energy efficiency, propulsion systems, alternative fuels for both passenger and freight transport and compensatory carbon removals. Details of our analytic approach are in Methods (Supplementary Figs. 3 and 4 and Supplementary Table 1 ). We develop and analyse a range of mid-century decarbonization scenarios for the aviation industry decomposing historical and future aviation emissions using a sector-specific variant of the Kaya identity:
where F represents fossil fuel CO 2 emissions from global aviation (neglecting life-cycle emissions of the aircraft and the supply chain of fuel), D is demand or distance flown and E is the energy consumed by flying aircraft, such that e is energy intensity of air transport and f is the carbon intensity of energy used for air transport. We analyse three pathways of demand ( D ) and energy intensity ( e ) based on ‘Business-as-usual’ (BAU), ‘Industry’ and ‘Ambitious’ projections and combine them with three pathways for carbon intensity ( f ), namely ‘Carbon intensive’, ‘Reduced fossil’ and ‘Net-zero’.
Demand for aviation
Total aviation demand in 2019 was almost 1 trillion ton-kilometer equivalent (tkm e or 11.1 trillion passenger-kilometer equivalent, pkm e ), with 78% representing passenger flights and 22% freight (Fig. 1a , black line). Travel advisories and border restrictions during the global pandemic led to a sharp decline in the air transport of passengers 7 , driving global demand down to about 0.45 trillion tkm e (5.0 trillion pkm e ) in 2020: 18% and 65% decreases in freight and passenger transport, respectively. Freight demand fully recovered in 2021 22 , but as of July 2022, passenger demand was still about 25% below pre-pandemic levels 23 . While ICAO estimates that it may be several more years before passenger demand recovers to 2019 levels, IATA projects a faster recovery of air travel to 2019 levels by 2023 24 . It is worth noting that demand varies regionally, with about 38%, 24% and 23% of passenger-kilometers being attributed to Asia and the Pacific, Europe and North America, respectively. Although the share of passenger demand is substantially smaller in the Middle East (9%), Latin America and the Caribbean (5%) and Africa (2%), demand in those regions has been rapidly increasing, for example, growing by 234% in the Middle East between 2007 and 2019 (Supplementary Fig. 5 ).
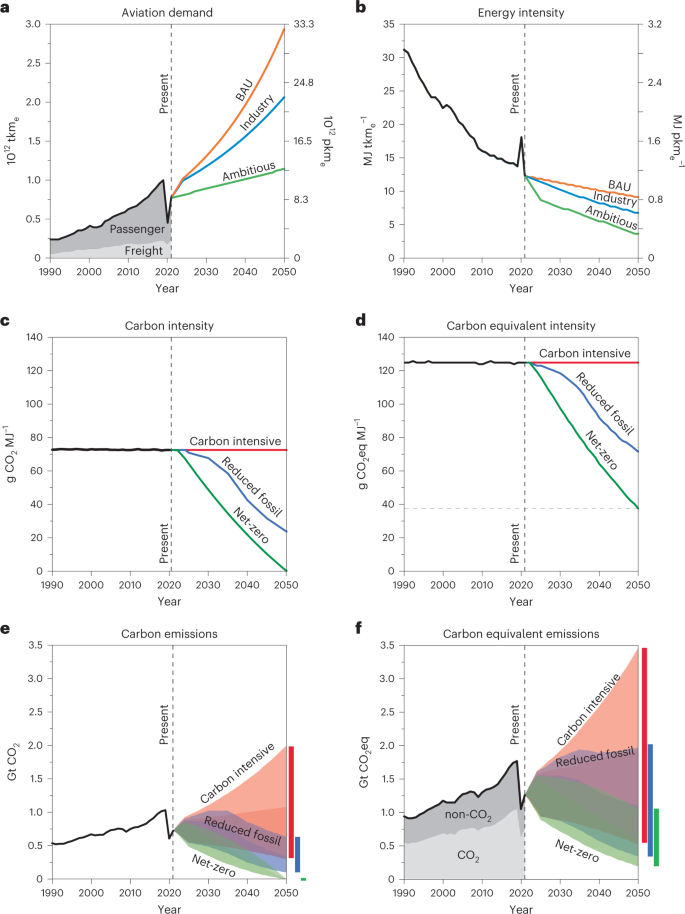
a , Total global aviation demand ( D ) for BAU (orange), Industry projections (blue) and Ambitious (green) scenarios. b , Energy intensity of air transport ( e ) for the same scenarios. c , Carbon intensity of aviation energy ( f ) in gCO 2 MJ −1 for Carbon Intensive (red), Reduced fossil (blue) and Net-zero (green) scenarios. d , Carbon equivalent intensity ( f ) for aviation in gCO 2 eq MJ −1 based on a GWP100 for the same scenarios. e , Carbon dioxide emissions in GtCO 2 from fossil jet fuel burning by combining three carbon intensity scenarios ( f ) with three demand and energy intensity scenarios (BAU D with BAU e , Industry D with Industry e and Ambitious D with Ambitious e ). f , Carbon-equivalent emissions in GtCO 2 eq based on a GWP100 estimate based on Lee et al. 6 . Historical data (black) for each panel are shown for 1990–2021; projections are shown for 2022–2050. Panel a shows the breakdown of total demand by passenger and freight aviation. Panels e and f represent the emissions ranges for each group of demand and energy-intensity scenarios in combination with the different carbon-intensity scenarios. Panel f shows the historical breakdown between CO 2 and non-CO 2 emissions. All scenario assumptions and sources are in Supplementary Table 1 . For other GWP and GTP, refer to Supplementary Fig. 9 .
Despite such short-term uncertainty, industry projections consistently anticipate continued growth in demand of air transport in the coming decades 8 , whereas other researchers have argued that substantial reductions in future demand are possible via behavioural changes and shifts to high-speed trains 4 . The demand scenarios in Fig. 1a thus span a wide range of trajectories: ‘BAU’ increases of 4% per year (to 2.9 trillion tkm e or 32.1 trillion pkm e in 2050; orange curve) 8 , ‘Industry’ projections of an average of 2.8% increase per year (2.1 trillion tkm e or 23.7 trillion pkm e ; blue curve) 8 and ‘Ambitious’ demand shifts that keep growth to an average of 1% per year (1.1 trillion tkm e or 12.4 trillion pkm e ; green curve) 25 . It should be noted that the Ambitious scenario implies a sudden and drastic divergence in the historical relationship between aviation demand and expected population and economic growth (Supplementary Fig. 1 ).
Energy intensity of aviation
The energy intensity of aircraft has declined by an average 1% per year since 1970 26 , falling from 31.6 MJ tkm e −1 (2.8 MJ pkm e −1 ) in 1990 to about 12.6 MJ tkm e −1 (1.1 MJ pkm e −1 ) in 2021 (Fig. 1b , black line). The spike in 2020 is driven by the global pandemic, when the rapid drop-in passenger demand led to decreases in the load factors of flights (that is, the share of seats filled) and thus increases in energy intensity (to 18.3 MJ tkm e −1 or 1.7 MJ pkm e −1 ). Improvements since 2010 reflect the release of fuel-efficient aircraft such as the Airbus A320neo and A350 and the Boeing 737 MAX and 787, but the International Council on Clean Transportation does not expect new aircrafts and thus substantial decreases in energy intensity in the next few years 26 . Despite this, the ICAO’s A40-18 resolution in 2019 set a goal of improving the fuel efficiency of international flights by 2% per year until 2050 14 . Even more ambitiously, a mid-century net-zero scenario developed by the IEA includes reductions in the energy intensity of international flights of an average 7% from 2019 to 2025, followed by a subsequent 2% yearly reduction to 2030 7 .
The scenarios shown in Fig. 1b span the full range of these future energy intensities, from ‘BAU’ reductions of 1% per year (to 9.4 MJ tkm e −1 or 0.85 MJ pkm e −1 in 2050; orange curve) 26 , ‘Industry’ reduction commitments of 2% per year (7.0 MJ tkm e −1 or 0.63 MJ pkm e −1 ; blue curve) 14 and ‘Ambitious’ reductions of an average of 4% per year (extrapolating the rapid decreases in the IEA net-zero scenario to reach 3.7 MJ tkm e −1 or 0.34 MJ pkm e −1 in 2050; green curve) 7 . Here again, it is not clear that the energy intensities in the most ambitious scenario are physically possible, but some studies have theorized that revolutionary improvements such as open rotors 27 , blended wing-body airframes 28 and hybridization 29 , and more efficient air traffic management, could bring important efficiency gains 25 .
Carbon intensity of energy for aviation
Historically, jet fuel (that is, fossil kerosene-based Jet A/A-1) has been the energy source for almost all commercial aircraft, resulting in a near-constant carbon intensity of ∼ 73.5 gCO 2 MJ −1 or 124.9 gCO 2 eq MJ −1 (including combustion emissions only; Fig. 1c , black curve). In recent years, some airlines have begun using bio-based jet fuel—which could decrease carbon intensity of aviation energy—but uptake has been slow: bio-based jet fuel production was about 140 million liters in 2019. This represented less than 1% of aviation fuel use in that year 30 and was mostly blended with fossil fuels based on standard D7566 from the ASTM, which allows a maximum 50% blend 31 . The first commercial demonstration plane using 100% biofuels flew on December 2021, and few have done it since 32 . Looking forward, industry groups nonetheless project rapid decreases in the carbon intensity of aviation energy. The International Renewable Energy Agency’s (IRENA) 1.5 °C scenario assumes that by mid-century, 70% of aviation’s energy demand is met by SAFs, while 14% comes from electricity and hydrogen 33 . Similarly, IATA’s net-zero commitment projects that 65% of 1.8 GtCO 2 (their estimated 2050 emissions) will be abated by using SAFs, with hydrogen and electricity-powered aircraft abating 13% (ref. 18 ). The IEA’s net-zero scenario includes 75% of all aviation energy demand being SAF by 2050 but with more modest deployment of electric planes 25 . It is worth noting that given their energy density, only short-haul flights (<3 hours) could be powered by electricity and hydrogen (Supplementary Fig. 6 ).
There are three carbon-intensity scenarios shown in Fig. 1c,d . First, a ‘Carbon intensive’ option that continues to rely on fossil jet fuel and thus maintains 73.5 gCO 2 MJ −1 or 124.9 gCO 2 eq MJ −1 (red curve, Supplementary Table 2 ). Second, a ‘Reduced fossil’ pathway in which 65% of energy demand by medium- and long-haul aviation in 2050 is met by SAFs (with 35% still met by fossil jet fuel) and 13%, 57% and 30% of short-haul aviation energy demand is met by non-emitting propulsion systems, SAFs and fossil jet fuel, respectively. This leads to 23.9 gCO 2 MJ −1 or 71.7 gCO 2 eq MJ −1 in 2050 (blue curve, Supplementary Table 3 ). And third, a ‘Net-zero’ pathway in which, by 2050, there is no combustion of fossil jet fuel. In this scenario, 100% of medium- and long-haul aviation energy in 2050 is supplied by SAFs, and 50% of short-haul fights are powered by other non-emitting propulsion systems, with the rest being SAFs. This leads to 0 gCO 2 MJ −1 or 37.6 gCO 2 eq MJ −1 by 2050 (green curve, Supplementary Table 4 ). Note that these scenarios assume that the combustion emissions from SAFs are net-zero with respect to atmospheric carbon and have the same non-CO 2 emissions as fossil fuels, assumptions we discuss in more detail below.
Aviation emissions
According to IEA estimates, aviation carbon emissions were 1.03 GtCO 2 in 2019 7 , 64% of which were related to international flights and 36% from domestic flights. Emissions plunged to 0.61 GtCO 2 in 2020 amid COVID-19 lockdowns and rebounded somewhat to 0.7 GtCO 2 in 2021 7 (Fig. 1e , black curve). On the basis of GWP100 6 , aviation’s total equivalent emissions in 2019 were about 1.7 GtCO 2 eq and dropped to 1.03 GtCO 2 eq in 2020 (Fig. 1f , black curve). Future emissions will reflect the combination of changes in demand, energy intensity of aviation and the carbon intensity of aviation energy, with important regional distinctions. By 2019, the United States represented ~28% of global aviation emissions, followed by China (10%) and larger European nations (18%) (Supplementary Fig. 5 ).
Combining our scenarios of demand and intensities as described in Supplementary Fig. 3 gives ranges of emissions trajectories shown in Fig. 1e,f . On the upper end, BAU growth in demand (that is, +4% per year) and improvements in energy intensity (that is, −1% per year), with continued use of fossil jet fuel leads to annual aviation emissions of 2.0 GtCO 2 (3.4 GtCO 2 eq) in 2050 (top of red shading in Fig. 1e,f ). At the other extreme, phasing out fossil jet fuel entirely would eliminate carbon aviation emissions by 2050 (green shading in Fig. 1e )—but might entail large cost increases (as discussed below). Accounting for non-CO 2 impacts, total equivalent emissions in such an ambitious scenario would be about 0.2 GtCO 2 eq by mid-century (Fig. 1f ). Notably, replacing 65% of medium- and long-haul aviation fossil jet fuel with SAFs could still result in annual carbon emissions of 0.65 GtCO 2 (higher than emissions in 2020) or about 1.9 GtCO 2 eq in 2050 under BAU changes in demand and energy intensity (top of blue shading in Fig. 1e,f ; Fig. 2d ). Accounting for the non-CO 2 impacts from aviation means the sector will not be zero emissions unless carbon dioxide removals (CDR) are included.
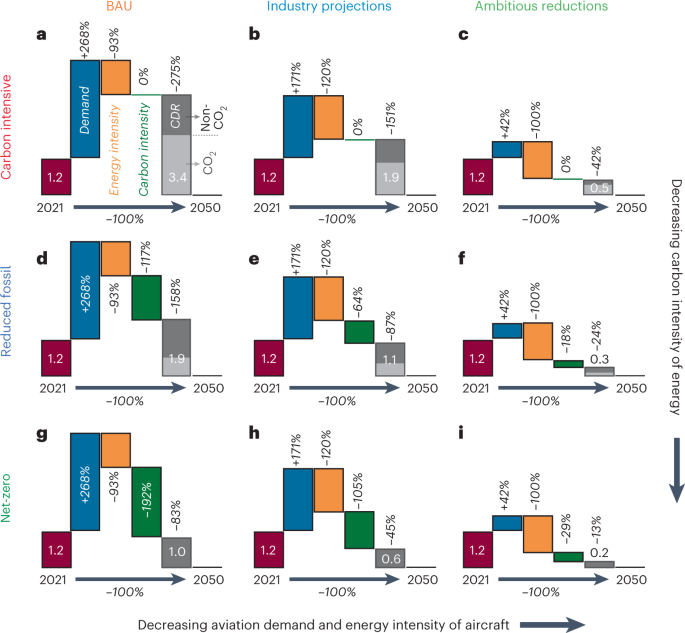
Each column represents a combination of demand and energy intensity ( De ), and each row represents a carbon-intensity trajectory ( f ). Each panel represents a demand and energy-intensity trajectory combined with a specific carbon intensity ( Def ). Colours for the headers represent low- (orange/red), medium- (blue) and high-ambition (green), for example, panel a represents the lowest ambition scenario with BAU demand and energy intensity and a carbon intensive fuel mix. Each bar within each panel represents a Kaya parameter: historical emissions in 2021 (maroon), increase in emissions based on projected demand (blue), decrease in emissions based on energy-intensity improvements (orange), potential further reductions due to changes in carbon intensity of energy (green) and carbon dioxide removals (CDR) needed to reach net-zero by 2050 (grey). The CDR grey bar is divided into two, representing the split between CO 2 and non-CO 2 equivalent emissions in each scenario.
Figure 2 reveals the relative contributions of different mitigation levers by comparing relative changes between 2021 and 2050 for aviation total climate impacts and the magnitude of CDR needed to achieve net-zero emissions. For example, annual emissions nearly triple assuming BAU changes (+175%), driven by surging demand for air transport (blue bar; Fig. 2a ), requiring the highest CDR to meet net-zero targets (3.4 GtCO 2 ), a removal that could cost up to a trillion dollars (Supplementary Fig. 7 ). In contrast, assuming somewhat lower increases in demand, an almost tripling of historical decreases in energy intensity and that two-thirds of fuel are sustainable and net-zero, annual emissions in 2050 could be roughly equivalent of what they were in 2021 (−13%; Fig. 2e ), with a need for CDR for 1.1 GtCO 2 . Finally, the decreases in carbon intensity of aviation energy in net-zero scenarios (green bar; Fig. 2g–i ) are heavily dependent on projected changes in aviation demand and energy intensity—the higher demand for air travel and the lower the improvements in energy intensity, the more important the share of SAFs. In turn, greater use of SAFs lowers the need for CDR to reach net-zero radiative forcing.
Sustainable aviation fuels
The quantity of SAFs required to meet net-zero goals is inversely proportional to changes in aviation demand and energy intensity (Fig. 3 ). Although this demand might also be reduced by using hydrogen or battery electric propulsion systems, the low energy density of such alternatives will probably limit their use to short-haul applications (Supplementary Fig. 6 ). For example, assuming a 60% fuel fraction (that is, the share of maximum take-off weight allocated to fuel), 90% increases in energy efficiency and 1,500 kWh t −1 H 2 , larger body aircraft such as a Boeing 777-200 or Airbus 380-800 (whose fuel fraction is ∼ 50%) converted to hydrogen propulsion would not be anywhere near able to cover the distance of common long-haul routes such as New York to London (5,500 km) or Los Angeles to Beijing (10,000 km). Similar estimates show that the range of large battery electric planes would be ∼ 500 km (Supplementary Fig. 6 ). Nonetheless, our Net-zero scenarios assume that half of short-haul flights might be serviced by hydrogen or battery electric planes (Supplementary Table 4 ).
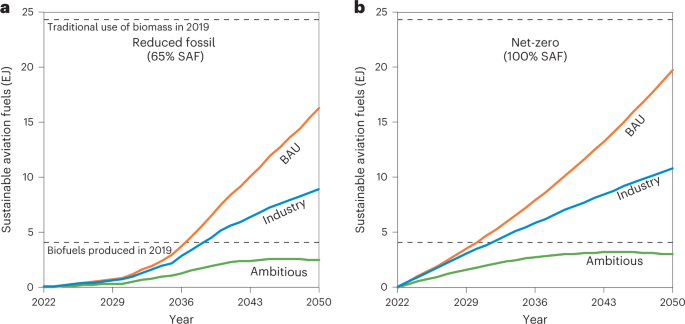
a , b , SAF demand varies considerably for reduced fossil ( a ) and net-zero ( b ) pathways. Each solid line represents a combination of demand ( D ) and energy intensity ( e ); orange stands for BAU, blue for Industry projections and green for Ambitious pathways. The dashed horizontal grey line in the bottom shows total biofuel production worldwide in 2019 81 , whereas the top dashed line shows the total global traditional use of biomass in the same year 34 . For reference, in 2019, total global bioenergy use was almost 64 EJ (ref. 34 ), while bio-jet fuel production was only 0.005 EJ (ref. 35 ).
Thus, Fig. 3 shows that without extreme reductions in aviation demand and energy intensity (that is, the green ‘Ambitious’ curves), by 2050, demand for SAFs in all of our scenarios is more than double the quantity of global production of biofuels in 2019 (~4 EJ including ethanol, biodiesel and hydrotreated vegetable oil) 34 and about 1,800 times more than the 0.005 EJ of bio-based jet fuel produced in 2019 35 . Such a biofuel demand could derive in a land expansion as high as 300 million hectares (~19% of global cropland in 2019; Supplementary Fig. 8 ). It is likely, however, that as electrification of other sectors continues, some of the ~64 EJ of global biomass energy supply 34 are diverted to produce bio-based jet fuels—and it is unlikely that the entirety of SAF demand is met by biofuels. In addition to biofuels, SAFs might ultimately include hydrocarbons produced by Fischer–Tropsch (FT) or methanol synthesis using carbon captured from the atmosphere and hydrogen generated without fossil CO 2 emissions (for example, by electrolysis using renewable or nuclear electricity) 36 .
Whether biofuels or synthetic fuels, a major barrier to the penetration of SAFs is cost, which, in turn, depends on the cost of feedstocks and the costs and efficiency of conversion processes. In the case of synthetic fuels, the cost of hydrogen primarily reflects electrolyser and electricity costs, and the cost of captured carbon depends on the technology involved. For example, assuming current costs of electrolytic hydrogen and captured carbon are around US$4.50 kg −1 H 2 (ref. 36 ) and US$0.25 kg −1 CO 2 (ref. 37 ), respectively, synthetic jet fuel costs are about US$2.60 l −1 , more than three times higher than the global 2022 average cost of fossil jet fuel (as of 31 May 2022) 38 (Fig. 4a ). If we incorporated the costs of removing carbon from the atmosphere to compensate for the non-CO 2 impacts embodied in burning one liter of synthetic jet fuel, then this cost would increase to about US$3.20 l −1 (based on a GWP100 and US$350 t −1 CO 2 ; Fig. 4b ). These estimates are broadly consistent with other recent studies that reported costs of synthetic fuel ranging from US$1.30 to US$4.70 per liter (refs. 39 , 40 ). Economies of scale and learning-by-doing may substantially reduce electrolyser and carbon capture costs in the future, making synthetic fuels more competitive 36 .
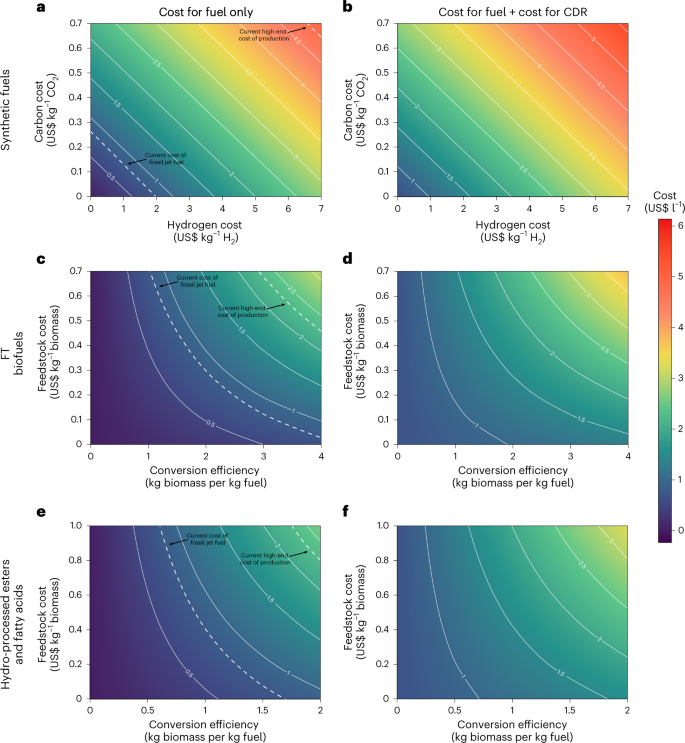
a – f , Contours show costs of synthetic fuel ( a , b ), FT biofuels ( c , d ) and hydro-processed esters and fatty acids ( e , f ) based on key input costs and conversion efficiencies. The left three panels ( a , c , e ) include the cost for producing each fuel. The right three panels ( b , d , f ) represent the same costs as in the right panels plus what it would cost to remove from the atmosphere the carbon equivalent non-CO 2 emissions embedded in a liter of SAF for a GWP100 and an assumed cost of CDR of US$350 t −1 CO 2 (for other assumptions, refer to Supplementary Fig. 7b ). For comparison, one of the dashed white lines in each left panel indicates the 2022 average cost of fossil jet fuel as of the end of May (US$0.80 l −1 ), according to IATA’s Fuel Price Monitor 38 . The other dashed white line represents upper-end costs from the literature 35 . Further details of calculations are in Methods and Supplementary Tables 6 – 11 .
Even though there are several conversion pathways for biofuels, FT biofuels and hydro-processed esters and fatty acids (HEFA) are among the few advanced biofuels with ‘near commercial’ fuel readiness level. Near-commercial readiness means the conversion pathway has been certified and the technology is beyond the research and development stage. On the basis of average feedstock costs of US$0–1.10 kg −1 of biomass and conversion efficiencies between 30–50% ( ∼ 2–4 kg biomass per kg fuel) 41 , current production costs for FT biofuels are between US$1.00 and US$2.30 l −1 (ref. 35 ) or US$1.68 and US$2.97 l −1 , accounting for the costs of removing the carbon equivalent to the non-CO 2 forcing in that liter of biofuel (Fig. 4c,d ). The lower end uses a zero-cost waste feedstock with 67% and 33% of the production cost represented by capital and operating expenditures, respectively; the upper end uses a lignocellulose feedstock that is 33% of production cost, with the remainder 45% and 22% represented by capital and operating expenses, respectively 35 . Although the low end of this range approaches the current cost of fossil jet fuel, the additional expense may be limiting uptake in a cost-competitive industry where, at least in the near-term, emissions reductions remain mostly voluntary. Achieving cost parity could thus greatly increase use of FT biofuels and might entail a carbon price of as little as US$78 t −1 CO 2 . For HEFA biofuels, costs of feedstocks (for example, from used cooking oil to jatropha oil) are routinely US$0.70–2.60 kg −1 (ref. 35 ) and unlikely to decrease much in the future. The HEFA conversion pathway has the highest efficiency compared with other bio-based jet fuel routes at around 76% (ref. 42 ) ( ∼ 1–2 kg biomass per kg fuel), with production cost ranges between US$0.80 and US$2.30 l −1 (ref. 35 ) or US$1.46 and US$3.00 l −1 if the non-CO 2 forcing was included (Fig. 4e,f ). Although the lower-end costs are less than fossil jet fuel, feedstock availability is limited as it represents used cooking oil that is a byproduct of consumption, and 90% of this feedstock is already used for biodiesel production (at least in the European Union) 35 .
Without ambitious reductions in air transport demand and improvements in aircraft energy efficiency, decarbonizing aviation will require important quantities of ‘drop-in’ sustainable aviation fuels (SAFs), especially given the number and long lifetime of commercial aircraft ( ∼ 23,000 and >25 years). As much as 19.8 EJ of SAFs—nearly five times the total quantity of biofuels produced worldwide in 2019 34 —might be necessary to achieve net-zero carbon emissions under business-as-usual changes in demand and energy intensity. Such scale would require the ethanol and biodiesel industries to grow four times faster than they did in the early 2000s 43 . Additionally, in a net-zero world, bio-based jet fuels would compete for feedstocks with other hard-to-decarbonize sectors and with electricity generation from bioenergy with carbon capture and storage (which would provide a source of negative emissions).
Because of aviation’s non-CO 2 forcing, achieving a net-zero emissions sector would also rely on CDR ranging from 0.2 to 3.4 GtCO 2 in our scenarios. If carbon credits are less expensive than SAFs, airlines may seek to offset rather than reduce their combustion emissions (Supplementary Fig. 7 ). Indeed, many airlines currently offer their customers offsets, and ICAO’s CORSIA establishes mandatory schemes to achieve carbon neutrality, relying mostly on offsets 14 . However, such credits are increasingly facing questions of permanence and additionality 44 that make reliable mitigation through fuel switching and operational shifts, such as contrail avoidance by plane rerouting, vital 45 .
Given that airline net profits in 2019 were about US$3.26 per thousand passenger-kilometers (ref. 46 ) and fuel represents between 20% and 30% of airlines’ operating costs 47 , the high current costs of SAFs (2–4 times higher than fossil jet fuel based on recent references; Fig. 4 ) may not be feasible. These high costs make fuel switching the most difficult in developing regions, where aviation demand is growing the fastest. Projected decreases in the costs of electrolytic hydrogen 36 and captured carbon 48 would make synthetic fuels more affordable, and higher conversion efficiencies and lower feedstock costs would help FT and HEFA biofuels. Such improvements may be induced via specific policy incentives such as cleaner aviation fuel tax credits (as those included in the Inflation Reduction Act in the United States) 49 and low-carbon fuel standards 50 , though HEFA feedstock costs have been quite volatile in recent years 51 . Carbon pricing could also change the incentive structure and make SAFs more competitive, potentially hastening deployment and further reducing costs via learning and economies of scale 35 .
Several important limitations and caveats apply to our findings. Although it is possible to produce SAFs with net-zero or even net-negative CO 2 emissions to the atmosphere, recent studies have estimated that the life-cycle emissions related to biofuels often entail emissions of 6–108 gCO 2 eq MJ −1 (ref. 3 ). ICAO’s SAF requirements only demand a 10% emissions reduction 14 , though we have assumed SAFs to be net-zero carbon. Ensuring the carbon neutrality of future biofuels will require resolving a host of complex accounting decisions, such as the time allowed between an emission and an uptake, the global warming potential of non-CO 2 and the attribution of emissions from indirect land-use change 52 , 53 . Moreover, the American Society for Testing Materials certification currently allows blends of up to 50%, mostly because of the low aromatic content of SAFs. Fully deploying SAFs would require allowing 100%, and although manufacturers such as Boeing have goals of achieving this by 2030, it is not yet guaranteed 31 , 54 . Additionally, the energy density of SAFs is less than that of fossil jet fuel, which could have implications for their value and aircraft range if fully deployed and derive in higher fuel consumption leading to higher non-CO 2 radiative forcing. Compared with 34.7–35.3 MJ l −1 of fossil jet fuel 55 , the energy densities of synthetic methanol, bioethanol, biodiesel and hydrotreated vegetable oil are 15.6 MJ l −1 , 21.4 MJ l −1 , 32.7 MJ l −1 and 34.4 MJ l −1 , respectively 56 , 57 . More generally, while we consider non-CO 2 emissions from aviation, much uncertainty remains on accounting for these emissions, particularly in terms of short-lived climate forcers such as contrails 58 . We assume that SAFs have the same non-CO 2 emissions as fossil jet fuels, though some studies have found that cleaner aviation fuels could both increase or decrease contrail formation 59 , 60 .
Despite these considerations, our analysis demonstrates the large-scale increases in SAF production that may be necessary to decarbonize the sector and the extent to which decreases in demand and improvements in energy intensity can reduce future demand for SAFs and the need for CDR. The main challenges to scaling up such sustainable fuel production include technology costs and process efficiencies, both of which are thus key targets for policies and innovation. Additionally, the interactions with food security, local communities and land use are enormous hurdles for such a ramp-up and come with their own increasingly difficult trade-offs. Yet with moderate growth in demand, continued improvements in aircraft energy efficiency and operational and infrastructure improvements, new propulsion systems for short-haul trips, greatly accelerated production of SAFs and the possibility of balancing non-CO 2 radiative forcing with equivalent amounts of CDR, the aviation sector could achieve net-zero emissions by 2050.
In this paper, we use the Kaya identity to decompose historical emissions from global aviation and to analyse future pathways for the decarbonization of the sector. This approach has been applied in other studies to analyse historical global and regional drivers of CO 2 emissions as a whole 61 and in specific sectors or regions for historical emissions and future trajectories 62 , 63 . We analyse emissions, energy and air travel demand data from the International Energy Agency (IEA) 7 , 25 , 64 , 65 , 66 , 67 , the Carbon Monitor 68 , the World Bank 69 , 70 , 71 , ICAO 8 , 72 , 73 , 74 and IATA 18 .
We develop a total of nine scenarios, shown in Supplementary Fig. 3 and Supplementary Fig. 4 , based on variations for demand and energy intensity ( De) and carbon intensity ( f) . The decomposition of the scenarios and sources for the data for each parameter and the future projected assumptions are available in Supplementary Table 1 .
Kaya Parameters
Given the uncertainty regarding the recovery of and future demand of air travel, we develop three demand-based scenarios with different projections. In the Business-as-usual scenario, passenger demand recovers by 2024, consistent with ICAO’s central recovery projection 75 (based on IATA, freight aviation has already recovered) 22 , and future projection follows historical GDP growth 76 (1980–2019) of 4% between 2024 and 2050. In the Industry projections scenario, demand also recovers by 2024 and then grows yearly at 2.9% and 2.6% for passenger and freight demand, respectively, consistent with ICAO’s low post-COVID demand scenario 8 . In the Ambitious reductions scenario, we assume that behavioural change and consumer preferences derive a slight 12% increase in demand by 2050 compared with 2019, similar with the IEA’s net-zero scenario for aviation 25 , which translates to a 1% yearly increase in total aviation demand from 2022 to 2050 (Supplementary Table 1 provides more details).
Energy intensity
We model three energy-intensity-based scenarios. In the Business-as-usual scenario, we follow a 1% energy-intensity reduction per year, consistent with the 1970–2019 average 26 . For the Industry projections scenario, we assume that ICAO’s A40-18 resolution of 2% yearly improvements in fuel efficiency is met both internationally and domestically 72 . For the Ambitious reductions scenario, we assume energy-intensity reductions similar to the IEA’s net-zero scenario, with intensities decreasing rapidly between 2022 and 2025 and more modest decreases between 2025 and 2050, with an overall average yearly decrease of 4% from 2022 to 2050 7 (Supplementary Table 1 ).
Carbon intensity
There are three carbon-intensity scenarios in this study. In the Carbon intensive scenario, we assume that fossil jet fuel continues to be the main energy source for aviation, consistent with historical record, which leads to a carbon intensity of 73.5 gCO 2 MJ −1 (refs. 66 , 77 ) or 124.9 gCO 2 eq MJ −1 (from tank to wake, excluding fuel production emissions) (Supplementary Table 2 ). We neglect life-cycle, ‘well-to-tank’ emissions because such emissions are thought to represent a small fraction of the total (for example, 14.3 gCO 2 MJ −1 ) (ref. 50 ) and because these emissions are, in theory, much easier to avoid than the direct emissions from aviation itself 78 . That is, aviation emissions are particularly difficult to abate because the high-energy-density liquid fuels are needed to power large, long-distance flights, but life-cycle emissions of fuels could be avoided by, for example, electrification of mining or drilling equipment and processing facilities. In the Reduced fossil scenario, we follow IATA’s net-zero carbon emissions pathway introduced in the 77th Annual General Meeting. On the basis of IATA’s proposition, by 2050, 65% of 2050 estimated emissions are mitigated with SAFs, and new technologies (electric planes and/or hydrogen) mitigate 13%, only allowing electric planes to deploy in short-haul flights, starting in 2025 with less than 1%, linearly increasing to 13% by 2050 18 . In our scenario, this derives in a carbon intensity that decreases from 73.5 gCO 2 MJ −1 (or 124.9 gCO 2 eq MJ −1 ) in 2021 to 23.9 gCO 2 MJ −1 (or 71.7 gCO 2 eq MJ −1 ) in 2050 (Supplementary Table 3 ). The Net-zero scenario follows a more aggressive deployment of both SAFs and new propulsion technologies by 2050, and we assume that the entirety of medium- and long-haul planes are powered with SAFs and that for short-haul aviation, the split is 50–50 between SAFs and new propulsion planes. In our scenario, this derives in a carbon intensity that decreases from 73.5 gCO 2 MJ −1 (or 124.9 gCO 2 eq MJ −1 ) in 2021 to 0 gCO 2 MJ −1 (or 37.6 gCO 2 eq MJ −1 ) in 2050 (Supplementary Table 4 ). We assume that biofuels and synthetic fuels are net-zero carbon fuels and that they have the same non-CO 2 emissions as fossil jet fuel, that the electricity to power short-haul planes comes from a renewable grid—and thus also has a net-zero carbon content—and that hydrogen is a product of electrolysis (Supplementary Table 1 ).
Non-CO 2 emissions
In this study, we calculate CO 2 emissions based on the Kaya identity presented in equation ( 1 ), considering the demand for aviation, the energy intensity of aviation and the carbon intensity of the energy used to power aviation. Non-CO 2 emissions are calculated based on multipliers from Lee et al. 6 (Supplementary Table 5 ). These emissions include contrail cirrus, nitrous oxides, soot emissions, sulfur dioxide and water vapour. We use a global warming potential of 100 years (GWP100) and report GWP of 20 and 50 years and global temperature potentials (GTP) of 20, 50 and 100 years in Supplementary Fig. 9 .
For scenarios with a carbon intensity following the Reduced carbon and Net-zero pathways, we assume that SAFs are net-zero in terms of carbon but that they have the same non-CO 2 emissions as fossil jet fuel given the uncertainty around non-CO 2 . Therefore, the net-zero carbon-intensity scenarios result in emissions in terms of carbon equivalence even though they are considered net-zero carbon. The carbon intensity for scenarios including non-CO 2 emissions measured in gCO 2 eq MJ −1 was calculated based on estimated total fuel consumption and CO 2 eq emissions.
Cost estimates
Synthetic fuels.
The cost estimate for synthetic fuels is based on the mass balance, estimated as:
We are assuming a conversion efficiency of 80%. We are representing costs in liters, assuming 0.8 kg of synthetic fuel in each liter. Capital and operation costs are not considered in the equation as they represent only a minor portion of the cost compared to the hydrogen and carbon costs 79 . The constants 0.4 and 3.14 are the weight of hydrogen and CO 2 needed to produce 1 ton of CH 2 (3H 2 + CO 2 → CH 2 + 2H 2 O). The values for Fig. 4 are depicted in Supplementary Tables 6 and 7 .
FT biofuels
The cost estimate of Fischer–Tropsch (FT) biofuels includes capital expenditure, operational expenditure, feedstock costs and efficiencies. The cost is estimated as:
We are representing costs in liters, assuming that there are 0.88 kg in each liter for HEFA fuel, based on biodiesel density (1 liter = 0.88 kg). The constants 0.07 and 0.12 represent the capital and operation costs without considering the biomass cost per input 80 . The values for Fig. 4 are depicted in Supplementary Tables 8 and 9 .

HEFA biofuel
The cost estimate of hydro-processed esters and fatty acids (HEFA) biofuels includes capital expenditure, operational expenditure, feedstock costs and efficiencies. The cost is estimated as:
We are representing costs in liters, assuming that there are 0.88 kg in each liter for HEFA fuel, based on biodiesel density (1 liter = 0.88 kg). The constants 0.17 and 0.34 represent the capital and operation costs without considering the biomass cost per input 80 . The values for Fig. 4 are depicted in Supplementary Tables 10 and 11 .
Reporting summary
Further information on research design is available in the Nature Portfolio Reporting Summary linked to this article.
Data availability
Data were compiled from open sources (except for aviation’s energy consumption), and the references are mentioned in Supplementary Table 1 . The open-source data are available at https://doi.org/10.5281/zenodo.7187059 . The only exception is the IEA proprietary data for aviation’s energy consumption 65 . Historical emissions are from IEA 66 and CMP 68 , while future emissions are calculated based on equation ( 1 ). Historical demand is from ICAO 73 , 74 , while freight demand is from the World Bank 69 and IATA 22 . Future aviation demand follows assumptions with data from the International Monetary Fund 76 , ICAO 8 and IEA 25 . Historical energy-intensity values were calculated based on demand data and fuel consumption data from IEA 65 . Future energy-intensity estimates follow assumptions from Zheng et al. 26 , ICAO 72 and IEA 7 . Historical carbon intensity is calculated with data from Bosch et al. 50 , and carbon equivalent intensity is calculated based on Lee et al. 6 . Future carbon intensities are calculated based on penetration of different SAFs and electric/hydrogen-powered planes.
Code availability
Data processing was done in Excel. The generation of Fig. 4 and Supplementary Fig. 7 of this manuscript were done in R version 4.1.0 and are available at https://github.com/CandeBergero/Code-Fig4-Net-zero-emissions-aviation.git .
IPCC Summary for Policymakers. In Climate Change 2022: Mitigation of Climate Change (eds Shukla, P.R. et al.) (Cambridge Univ. Press, 2022).
Höhne, N. et al. Wave of net zero emission targets opens window to meeting the Paris Agreement. Nat. Clim. Change 11 , 820–822 (2021).
Article Google Scholar
Vardon, D. R., Sherbacow, B. J., Guan, K., Heyne, J. S. & Abdullah, Z. Realizing ‘net-zero-carbon’ sustainable aviation fuel. Joule 6 , 16–21 (2022).
Sharmina, M. et al. Decarbonising the critical sectors of aviation, shipping, road freight and industry to limit warming to 1.5–2 °C. Clim. Policy 21 , 455–474 (2021).
Klöwer, M. et al. Quantifying aviation’s contribution to global warming. Environ. Res. Lett. 16 , 104027 (2021).
Lee, D. S. et al. The contribution of global aviation to anthropogenic climate forcing for 2000 to 2018. Atmos. Environ. 244 , 117834 (2021).
Article CAS Google Scholar
Aviation (IEA, 2022); https://www.iea.org/reports/aviation
ICAO Appendix A: Traffic Forecasts (ICAO, 2021); https://www.icao.int/sustainability/Documents/Post-COVID-19%20forecasts%20scenarios%20tables.pdf
Gössling, S., Humpe, A., Fichert, F. & Creutzig, F. COVID-19 and pathways to low-carbon air transport until 2050. Environ. Res. Lett. 16 , 034063 (2021).
Schubert, I., Sohre, A. & Ströbel, M. The role of lifestyle, quality of life preferences and geographical context in personal air travel. J. Sustain. Tour. 28 , 1519–1550 (2020).
Higham, J. & Font, X. Decarbonising academia: confronting our climate hypocrisy. J. Sustain. Tour. 28 , 1–9 (2020).
Boeing Commits to Deliver Commercial Airplanes Ready to Fly on 100% Sustainable Fuels (Boeing, 2021); https://investors.boeing.com/investors/investor-news/press-release-details/2021/Boeing-Commits-to-Deliver-Commercial-Airplanes-Ready-to-Fly-on-100-Sustainable-Fuels/default.aspx
Airbus Reveals New Zero-Emission Concept Aircraft (Airbus, 2020); https://www.airbus.com/en/newsroom/press-releases/2020-09-airbus-reveals-new-zero-emission-concept-aircraft
Climate Change Mitigation: CORSIA Introduction to CORSIA Ch. 6 (ICAO, 2019); https://www.icao.int/environmental-protection/CORSIA/Documents/ICAO%20Environmental%20Report%202019_Chapter%206.pdf
United States: 2021 Aviation Climate Action Plan (Federal Aviation Administration, 2021); https://www.faa.gov/sites/faa.gov/files/2021-11/Aviation_Climate_Action_Plan.pdf
Report from the Commission to the European Parliament and the Council: Updated Analysis of the Non-CO 2 Climate Impacts of Aviation and Potential Policy Measures Pursuant to EU Emissions Trading System Directive Article 30(4) (European Union Aviation Safety Agency, 2020); https://eur-lex.europa.eu/legal-content/EN/TXT/?uri=SWD:2020:277:FIN
Beevor, J. & Alexander, K. Missed Targets: A Brief History of Aviation Climate Targets (produced by Green Gumption for Possible, 2022); https://www.wearepossible.org/our-reports-1/missed-target-a-brief-history-of-aviation-climate-targets
Net-Zero Carbon Emissions by 2050 (IATA, 2021); https://www.iata.org/en/pressroom/pressroom-archive/2021-releases/2021-10-04-03/
Gonzalez-Garay, A. et al. Unravelling the potential of sustainable aviation fuels to decarbonise the aviation sector. Energy Environ. Sci. 15 , 3291–3309 (2022).
Dray, L. et al. Cost and emissions pathways towards net-zero climate impacts in aviation. Nat. Clim. Change 12 , 956–962 (2022).
Becattini, V., Gabrielli, P. & Mazzotti, M. Role of carbon capture, storage, and utilization to enable a net-zero-CO 2 -emissions aviation sector. Ind. Eng. Chem. Res. 60 , 6848–6862 (2021).
Kulisch, E. IATA forecasts 2021 air cargo revenues to hit record $175B. FreightWaves (4 October 2021).
July Passenger Demand Remains Strong (IATA, 2022); https://www.iata.org/en/pressroom/2022-releases/2022-09-07-02/
Annual Review 2021 (IATA, 2021); https://www.iata.org/contentassets/c81222d96c9a4e0bb4ff6ced0126f0bb/iata-annual-review-2021.pdf
Net Zero by 2050 A Roadmap for the Global Energy Sector (IEA, 2021); https://iea.blob.core.windows.net/assets/deebef5d-0c34-4539-9d0c-10b13d840027/NetZeroby2050-ARoadmapfortheGlobalEnergySector_CORR.pdf
Zheng, X. S. & Rutherford, D. Fuel Burn of New Commercial Jet Aircraft: 1960 to 2019 (ICCT, 2020); https://theicct.org/sites/default/files/publications/Aircraft-fuel-burn-trends-sept2020.pdf
Hendricks, E. S. & Tong, M. T. Performance and weight estimates for an advanced open rotor engine. In 48th AIAA/ASME/SAE/ASEE Joint Propulsion Conference and Exhibit 2012 https://doi.org/10.2514/6.2012-3911 (2012).
Brown, M. & Vos, R. Conceptual design and evaluation of blended-wing-body aircraft. In AIAA Aerospace Sciences Meeting, 2018 https://doi.org/10.2514/6.2018-0522 (2018).
Nishizawa, A., Kallo, J., Garrot, O. & Weiss-Ungethüm, J. Fuel cell and Li-ion battery direct hybridization system for aircraft applications. J. Power Sources 222 , 294–300 (2013).
Sustainable Aviation Fuels Guide (ICAO, 2019); https://www.icao.int/environmental-protection/knowledge-sharing/Docs/Sustainable%20Aviation%20Fuels%20Guide_vf.pdf
Fact Sheet 2: Sustainable Aviation Fuel: Technical Certification (IATA, 2020); https://www.iata.org/contentassets/d13875e9ed784f75bac90f000760e998/saf-technical-certifications.pdf
From the Lab to the Sky: Five Things to Know about Biofuel-Powered Flights (DOE, 2022); https://www.energy.gov/eere/articles/lab-sky-five-things-know-about-biofuel-powered-flights
World Energy Transitions Outlook 2021 (IRENA, 2021); https://www.irena.org/-/media/Files/IRENA/Agency/Publication/2021/Jun/IRENA_World_Energy_Transitions_Outlook_2021.pdf
Bioenergy–Analysis (IEA, 2022); https://www.iea.org/reports/bioenergy
Reaching Zero with Renewables: Biojet Fuels (IRENA, 2021); https://www.irena.org/-/media/Files/IRENA/Agency/Publication/2021/Jul/IRENA_Reaching_Zero_Biojet_Fuels_2021.pdf
Ueckerdt, F. et al. Potential and risks of hydrogen-based e-fuels in climate change mitigation. Nat. Clim. Change 11 , 384–393 (2021).
Baylin-Stern, A. & Berghout, N. Is Carbon Capture Too Expensive? (IEA, 2021); https://www.iea.org/commentaries/is-carbon-capture-too-expensive
Fuel Price Monitor (IATA, 2022); https://www.iata.org/en/publications/economics/fuel-monitor/
Li, X. et al. Greenhouse gas emissions, energy efficiency, and cost of synthetic fuel production using electrochemical CO 2 conversion and the Fischer–Tropsch process. Energy Fuels 30 , 5980–5989 (2016).
Searle, S. & Christensen, A. Decarbonization Potential Of Electrofuels In The European Union (ICCT, 2018); https://theicct.org/publication/decarbonization-potential-of-electrofuels-in-the-european-union/
Leibbrandt, N. H., Aboyade, A. O., Knoetze, J. H. & Görgens, J. F. Process efficiency of biofuel production via gasification and Fischer–Tropsch synthesis. Fuel 109 , 484–492 (2013).
Doliente, S. S. et al. Bio-aviation fuel: a comprehensive review and analysis of the supply chain components. Front. Energy Res. 8 , 110 (2020).
Staples, M. D., Malina, R., Suresh, P., Hileman, J. I. & Barrett, S. R. H. Aviation CO 2 emissions reductions from the use of alternative jet fuels. Energy Policy 114 , 342–354 (2018).
Warnecke, C., Schneider, L., Day, T., La Hoz Theuer, S. & Fearnehough, H. Robust eligibility criteria essential for new global scheme to offset aviation emissions. Nat. Clim. Change 9 , 218–221 (2019).
Teoh, R., Schumann, U. & Stettler, M. E. J. Beyond contrail avoidance: efficacy of flight altitude changes to minimise contrail. Aerospace 7 , 121 (2020).
IATA Economics’ Chart of the Week (IATA, 2019); https://www.iata.org/en/iata-repository/publications/economic-reports/airline-profit-per-passenger-not-enough-to-buy-a-big-mac-in-switzerland/
Holladay, J., Abdullah, Z. & Heyne, J. Sustainable Aviation Fuel: Review of Technical Pathways (DOE, 2020); https://www.energy.gov/sites/prod/files/2020/09/f78/beto-sust-aviation-fuel-sep-2020.pdf
McQueen, N. et al. A review of direct air capture (DAC): scaling up commercial technologies and innovating for the future. Prog. Energy 3 , 032001 (2021).
H.R. 5376: Inflation Reduction Act of 2022 US House bill (2022).
Bosch, J., de Jong, S., Hoefnagels, R. & Slade, R. Aviation Biofuels: Strategically Important, Technically Achievable, Tough to Deliver (Grantham Institute, 2017); https://www.imperial.ac.uk/media/imperial-college/grantham-institute/public/publications/briefing-papers/BP-23-Aviation-Biofuels.pdf
Tao, L., Milbrandt, A., Zhang, Y. & Wang, W. C. Techno-economic and resource analysis of hydroprocessed renewable jet fuel. Biotechnol. Biofuels 10 , 261 (2017).
Land Sector and Removals Initiative (Greenhouse Gas Protocol, 2022).
Ocko, I. B. et al. Unmask temporal trade-offs in climate policy debates. Science 356 , 492–493 (2017).
van Dyk, S. Sustainable aviation fuels are not all the same and regular commercial use of 100% SAF is more complex. Green Air (1 February 2022).
Greenbaum, W. Alternative Jet Fuels. Stanford Univ. (2012); http://large.stanford.edu/courses/2012/ph240/greenbaum1/
Renewables 2018 Global Status Report (REN21, 2018); https://www.ren21.net/gsr-2018/pages/units/units/
Patterson, B. D. et al. Renewable CO 2 recycling and synthetic fuel production in a marine environment. Proc. Natl Acad. Sci. U.S.A. 116 , 12212–12219 (2019).
Cain, M. et al. Improved calculation of warming-equivalent emissions for short-lived climate pollutants. npj Clim. Atmos. Sci. 2 , 29 (2019).
Voigt, C. et al. Cleaner burning aviation fuels can reduce contrail cloudiness. Commun. Earth Environ. 2 , 114 (2021).
Caiazzo, F., Agarwal, A., Speth, R. L. & Barrett, S. R. H. Impact of biofuels on contrail warming. Environ. Res. Lett. 12 , 114013 (2017).
Raupach, M. R. et al. Global and regional drivers of accelerating CO 2 emissions. Proc. Natl Acad. Sci. U.S.A. 104 , 10288–10293 (2007).
Ma, M. & Cai, W. What drives the carbon mitigation in Chinese commercial building sector? Evidence from decomposing an extended Kaya identity. Sci. Total Environ. 634 , 884–899 (2018).
Mavromatidis, G., Orehounig, K., Richner, P. & Carmeliet, J. A strategy for reducing CO 2 emissions from buildings with the Kaya identity–a Swiss energy system analysis and a case study. Energy Policy 88 , 343–354 (2016).
Aviation–Fuels & Technologies (IEA, 2022); https://www.iea.org/fuels-and-technologies/aviation
IEA Oil Demand by Product for Non-OECD Countries , IEA World Energy Statistics and Balances (IEA, 2022); https://doi.org/10.1787/data-00511-en
IEA Detailed CO 2 estimates, IEA CO 2 Emissions from Fuel Combustion Statistics: Greenhouse Gas Emissions from Energy (IEA, 2022); https://doi.org/10.1787/data-00429-en
IEA Energy Intensity of Passenger Aviation in the Net Zero Scenario, 2000–2030—Charts—Data & Statistics (IEA, 2021); https://www.iea.org/data-and-statistics/charts/energy-intensity-of-passenger-aviation-in-the-net-zero-scenario-2000-2030
Carbon Monitor Project CO 2 Emissions (Carbon Monitor Project, 2022); https://carbonmonitor.org/international-aviation
World Bank Air Transport, Freight (million ton-km) (World Bank, 2022); https://data.worldbank.org/indicator/IS.AIR.GOOD.MT.K1
World Bank Population, Total (World Bank, 2022); https://data.worldbank.org/indicator/SP.POP.TOTL
World Bank GDP Per Capita (Current US$) (World Bank, 2022); https://data.worldbank.org/indicator/ny.gdp.pcap.cd
Resolution A40-18: Consolidated Statement of Continuing ICAO Policies and Practices Related to Environmental Protection–Climate Change (ICAO, 2019).
The Air Transport Industry (ICAO, 2014); https://www.icao.int/sustainability/documents/AirTransport-figures.pdf
The World of Air Transport in 2019 (ICAO, 2020); https://www.icao.int/annual-report-2019/Pages/the-world-of-air-transport-in-2019.aspx
Table A: COVID-19 Forecast Scenario Assumption Matrices (ICAO, 2020); https://www.icao.int/sustainability/Documents/post%20covid%20forecasts%20scenarios%20tables.pdf
IMF World Economic Outlook Database (IMF, 2021); https://www.imf.org/en/Publications/WEO/weo-database/2021/October
OECD.Stat WORLD_BBL.IVT (OECD, 2022); https://stats.oecd.org/BrandedView.aspx?oecd_bv_id=enestats-data-en&doi=data-00510-en#
Griffiths, S., Sovacool, B. K., Kim, J., Bazilian, M. & Uratani, J. M. Decarbonizing the oil refining industry: a systematic review of sociotechnical systems, technological innovations, and policy options. Energy Res. Soc. Sci. 89 , 102542 (2022).
Gielen, D., Castellanos, G. & Crone, K. The outlook for Powerfuels in aviation, shipping. Energy Post https://energypost.eu/the-outlook-for-powerfuels-in-aviation-shipping/ (2020).
Innovation Outlook: Advanced Liquid Biofuels (IRENA, 2016); https://www.irena.org/-/media/Files/IRENA/Agency/Publication/2016/IRENA_Innovation_Outlook_Advanced_Liquid_Biofuels_2016.pdf
IEA Global Biofuel Production in 2019 and Forecast to 2025–Charts–Data & Statistics (IEA, 2020); https://www.iea.org/data-and-statistics/charts/global-biofuel-production-in-2019-and-forecast-to-2025
Download references
Acknowledgements
C.B. and S.J.D. were supported by the US National Science Foundation and US Department of Agriculture (INFEWS grant EAR 1639318).
Author information
Authors and affiliations.
Department of Earth System Science, University of California, Irvine, Irvine, CA, USA
Candelaria Bergero & Steven J. Davis
Division of Economics and Business, Colorado School of Mines, Golden, CO, USA
Greer Gosnell
Innovation and Technology Center, International Renewable Energy Agency, Bonn, Germany
Dolf Gielen & Seungwoo Kang
The Payne Institute for Public Policy, Colorado School of Mines, Golden, CO, USA
Morgan Bazilian
Department of Civil and Environmental Engineering, University of California, Irvine, Irvine, CA, USA
Steven J. Davis
You can also search for this author in PubMed Google Scholar
Contributions
C.B. and S.J.D. conceived the study. C.B. performed the analyses with support from G.G., D.G., S.K., M.B. and S.J.D. The writing of the manuscript was done by C.B. and S.J.D., with inputs and revisions from G.G., D.G., S.K. and M.B.
Corresponding authors
Correspondence to Candelaria Bergero or Steven J. Davis .
Ethics declarations
Competing interests.
The authors declare no competing interests.
Peer review
Peer review information.
Nature Sustainability thanks Paolo Gabrielli and the other, anonymous, reviewer for their contribution to the peer review of this work.
Additional information
Publisher’s note Springer Nature remains neutral with regard to jurisdictional claims in published maps and institutional affiliations.
Supplementary information
Supplementary information.
Supplementary Tables 1–11 and Figs. 1–9.
Reporting Summary.
Rights and permissions.
Springer Nature or its licensor (e.g. a society or other partner) holds exclusive rights to this article under a publishing agreement with the author(s) or other rightsholder(s); author self-archiving of the accepted manuscript version of this article is solely governed by the terms of such publishing agreement and applicable law.
Reprints and permissions
About this article
Cite this article.
Bergero, C., Gosnell, G., Gielen, D. et al. Pathways to net-zero emissions from aviation. Nat Sustain 6 , 404–414 (2023). https://doi.org/10.1038/s41893-022-01046-9
Download citation
Received : 18 July 2022
Accepted : 07 December 2022
Published : 30 January 2023
Issue Date : April 2023
DOI : https://doi.org/10.1038/s41893-022-01046-9
Share this article
Anyone you share the following link with will be able to read this content:
Sorry, a shareable link is not currently available for this article.
Provided by the Springer Nature SharedIt content-sharing initiative
This article is cited by
Recent progress on the hydrodeoxygenation of lignin-derived pyrolysis oil using ru-based catalysts.
- Hyungjoo Kim
- Jae Hyun Park
- Do Heui Kim
Korean Journal of Chemical Engineering (2024)
Can industrial structure optimization and industrial structure transition both lead to carbon lock-in mitigation? The case of China
- Congyu Zhao
Environmental Science and Pollution Research (2024)
How to make climate-neutral aviation fly
- Romain Sacchi
- Viola Becattini
- Marco Mazzotti
Nature Communications (2023)
Carbon-neutral power system enabled e-kerosene production in Brazil in 2050
- Karl-Kiên Cao
- Patrick Jochem
Scientific Reports (2023)
Quick links
- Explore articles by subject
- Guide to authors
- Editorial policies
Sign up for the Nature Briefing newsletter — what matters in science, free to your inbox daily.

Climate Matters • December 6, 2023
Climate Change is Disrupting Air Travel
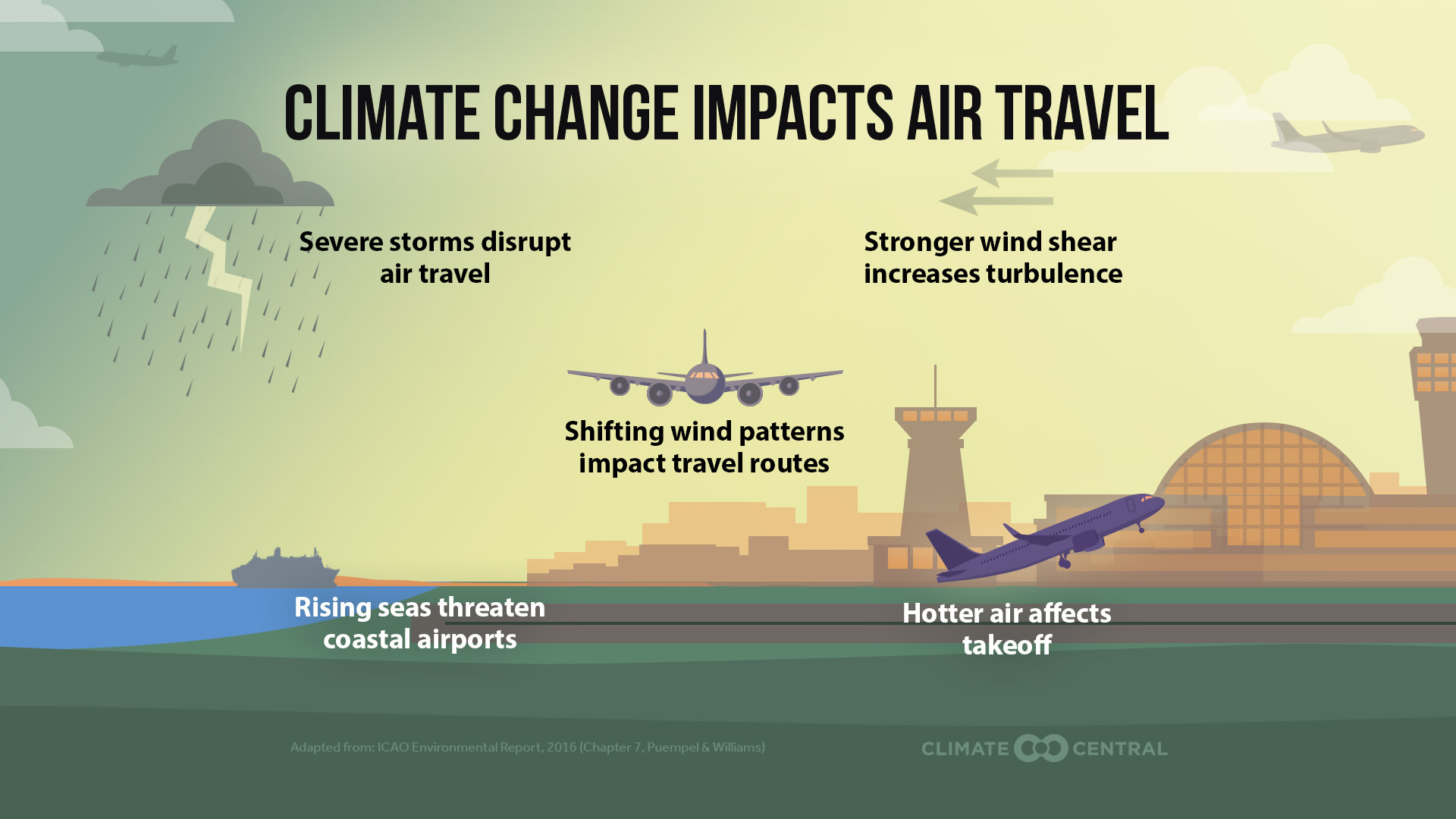
Click to download custom graphics
KEY CONCEPTS
Burning fossil fuels for aviation contributes to global warming — and the resulting warming is disrupting air travel.
Flooding caused by rising seas and storm surges threatens access and operations at coastal airports.
More extreme weather events, warmer air temperatures, and shifts in the jet stream can also disrupt air travel and increase in-flight safety risks.
Last year, 917 million passengers took over 15 million U.S.-based flights — an average of more than 42,000 flights per day.
Fossil fuels burned for global air travel and airport operations contribute to the carbon pollution that is warming the planet. In 2022, global air travel emitted more than 780 Mt CO2 , accounting for about 2% of global energy-related CO2 emissions that year. Emissions from aviation have steadily increased, quadrupling from 1966 to 2018.
Air travel not only contributes to heat-trapping pollution — the resulting warming now poses new and growing challenges for air travel.
Weather currently causes more than 75% of air traffic delays in the U.S. As climate change worsens coastal flooding and extreme weather events, more flights could be grounded from weather-related delays. A warming atmosphere can also increase in-flight turbulence.
Five ways climate change is disrupting air travel
1. Coastal airports are at risk from rising seas and storm surges.
Rising seas due to human-caused warming are worsening coastal floods during both regular high tides and coastal storms; and storm surge is affecting larger areas in many U.S. cities because of rising seas.
Runways at some major airports in U.S. cities and abroad are at risk of closures, delays, and damage due to coastal flooding, particularly after major storms . Access for passengers and employees can also be impacted if access roads are inundated.
2. Heat can affect plane capacity and restrict takeoff.
Warm air is less dense than cool air. Hotter temperatures at ground level therefore make it more difficult for airplanes to gain enough lift to take flight.
Hotter temperatures can cause weight restrictions for flight take-off — meaning fewer passengers and reduced capacity for luggage, cargo, and fuel. In some cases, planes may require longer runway distances to generate enough lift.
3. Climate change is increasing the risk of lightning strikes in flight.
The potential for severe storms is increasing in some parts of the U.S., particularly in the eastern half of the country. One study projects that annual lightning strikes in the U.S. could increase by 12% for every 1°C (1.8°F) of global warming.
Lightning strikes can damage electrical systems and equipment on large commercial aircraft. The average passenger plane is struck by lightning one or two times per year . Following lightning strikes, planes are subject to inspection and repairs, which can take them out of service and cause delays.
4. A shifting jet stream could mean a longer round trip journey.
In recent decades, scientists have observed changes in the jet stream — narrow bands of strong wind high in the atmosphere that move west to east along the boundaries between hot and cold air. The influence of global warming on these observed changes is not yet fully understood.
Research suggests that changing wind patterns could impact travel times in the Northern Hemisphere — potentially making west-bound flights longer, while speeding up east-bound flights. These changes could affect route planning, scheduling, and fuel consumption.
5. Increased wind shear in the jet stream is causing more hazardous turbulence.
One observed change to the jet stream includes stronger wind shear at flight cruising altitudes, which can increase turbulence during flights.
A certain type of turbulence known as clear-air turbulence can’t be seen by pilots or detected by radar. Clear-air turbulence is more likely to occur during winter months.
A recent study found a 41% increase in severe clear-air turbulence over the U.S. between 1979 and 2020 — and it is projected to increase further due to climate change.
The future of flying
Reducing heat-trapping pollution from air travel now is critical to limit future warming. But some consequences from rising temperatures are unavoidable, even with rapid cuts to carbon pollution. The aviation industry is faced with a need to adapt air travel for a warming world to protect passengers and employees, as well as keep costs under control.
Adaptation measures, including seawalls or other coastal defenses , can help protect existing airports from rising seas and storm surges, but they can be costly and complicated. The Shoreline Protection Program at San Francisco Airport is one example of how U.S. airports might build climate resilience.
In especially hot locations or during summer, airports may choose to schedule flights during cooler parts of the day to mitigate heat effects. Where possible, airports may expand runways to accommodate longer takeoff distances.
LOCAL STORY ANGLES
Find out if your local airport is vulnerable to coastal flooding..
Read Climate Central’s assessment of 23 major U.S. cities that are vulnerable to coastal flooding during this decade. Or use Climate Central’s Coastal Risk Screening Tool to map localized vulnerability to sea level rise and storm surges around your city’s airport.
See which airports are experiencing delays.
Extreme weather events, heat, or flooding can ground planes and disrupt domestic and international air travel with cascading impacts. The Federal Aviation Administration provides status updates on delays at airports across the U.S. (but specific flight information and delay causes aren’t reported here.)
CONTACT EXPERTS
Paul D. Williams, PhD Professor of Atmospheric Science. University of Reading Relevant expertise: atmospheric turbulence, jet streams, climate change, and aviation Contact: [email protected] * Available for interviews on or after December 11, 2023
FIND EXPERTS
Submit a request to SciLine from the American Association for the Advancement of Science or to the Climate Data Concierge from Columbia University. These free services rapidly connect journalists to relevant scientific experts.
Browse maps of climate experts and services at regional NOAA, USDA, and Department of the Interior offices.
Explore databases such as 500 Women Scientists , BIPOC Climate and Energy Justice PhDs , and Diverse Sources to find and amplify diverse expert voices.
Reach out to your State Climate Office or the nearest Land-Grant University to connect with scientists, educators, and extension staff in your local area.
The aviation sector wants to reach net zero by 2050. How will it do it?
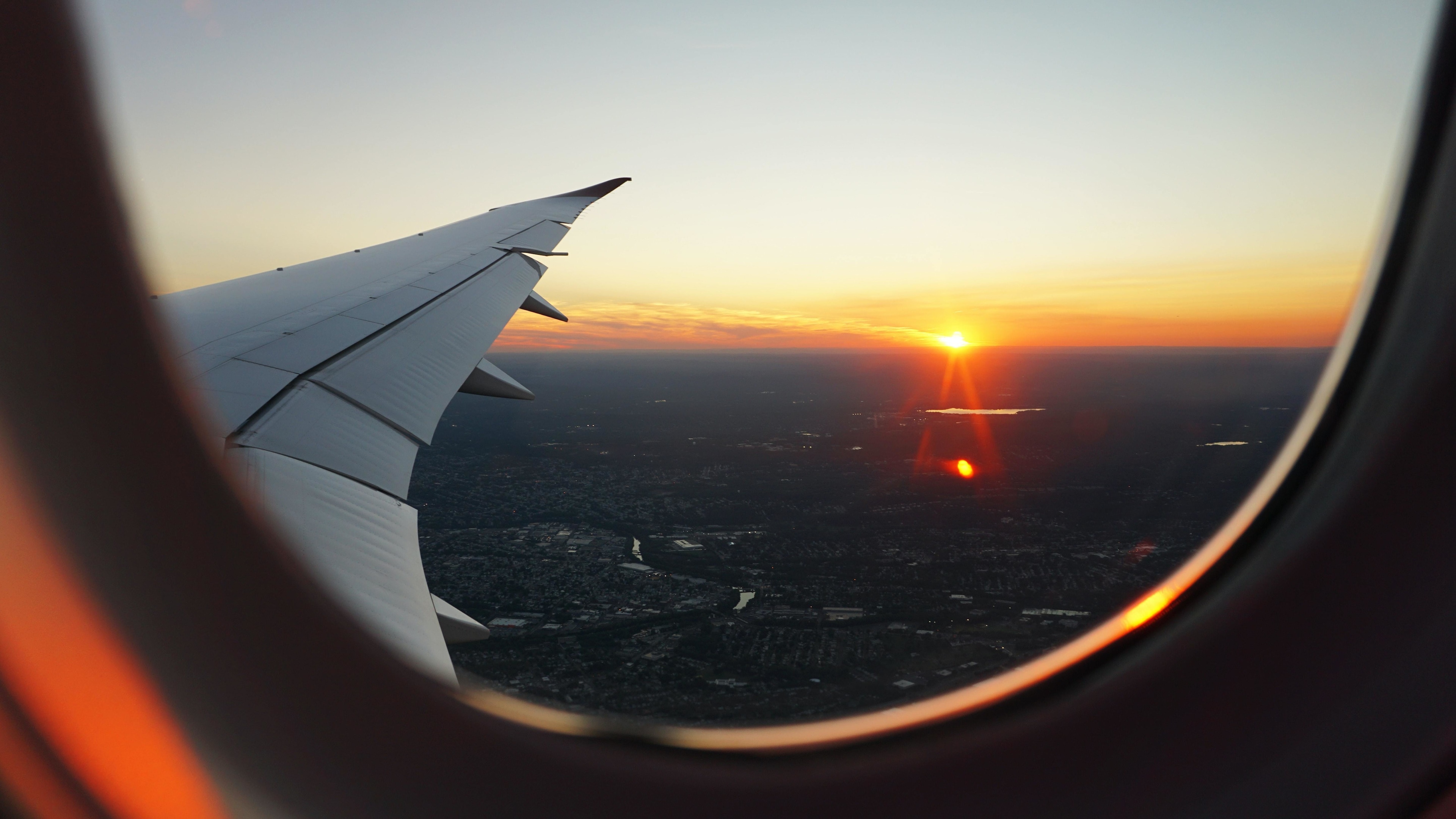
The global aviation industry has agreed to try to achieve net-zero emissions by 2050. Image: Unsplash/evadarron
.chakra .wef-1c7l3mo{-webkit-transition:all 0.15s ease-out;transition:all 0.15s ease-out;cursor:pointer;-webkit-text-decoration:none;text-decoration:none;outline:none;color:inherit;}.chakra .wef-1c7l3mo:hover,.chakra .wef-1c7l3mo[data-hover]{-webkit-text-decoration:underline;text-decoration:underline;}.chakra .wef-1c7l3mo:focus,.chakra .wef-1c7l3mo[data-focus]{box-shadow:0 0 0 3px rgba(168,203,251,0.5);} Stefan Ellerbeck
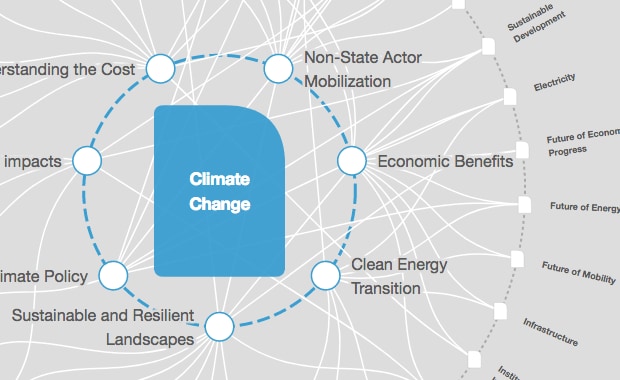
.chakra .wef-9dduvl{margin-top:16px;margin-bottom:16px;line-height:1.388;font-size:1.25rem;}@media screen and (min-width:56.5rem){.chakra .wef-9dduvl{font-size:1.125rem;}} Explore and monitor how .chakra .wef-15eoq1r{margin-top:16px;margin-bottom:16px;line-height:1.388;font-size:1.25rem;color:#F7DB5E;}@media screen and (min-width:56.5rem){.chakra .wef-15eoq1r{font-size:1.125rem;}} Climate Crisis is affecting economies, industries and global issues
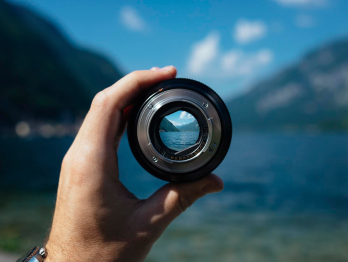
.chakra .wef-1nk5u5d{margin-top:16px;margin-bottom:16px;line-height:1.388;color:#2846F8;font-size:1.25rem;}@media screen and (min-width:56.5rem){.chakra .wef-1nk5u5d{font-size:1.125rem;}} Get involved with our crowdsourced digital platform to deliver impact at scale
Stay up to date:, climate crisis.
Listen to the article
- The global aviation industry has agreed to try to achieve net-zero emissions by 2050.
- The airline association IATA says using sustainable fuels and carbon offsetting will contribute more than 80% of the reduction in emissions.
- Other low-emission technologies like electric and hydrogen-powered aircraft are also being developed by major aviation players like Airbus.
For many of us, the ready availability of low-cost air travel has become the norm rather than the exception.
Yet aviation is responsible for around 2.5% of global CO2 emissions , with most aircraft powered by jet gasoline. The European Commission predicts that by the middle of the 21st century, demand for flying could increase aviation’s greenhouse gas emissions by upwards of 300% over 2005 levels if no drastic measures are taken to reduce them.
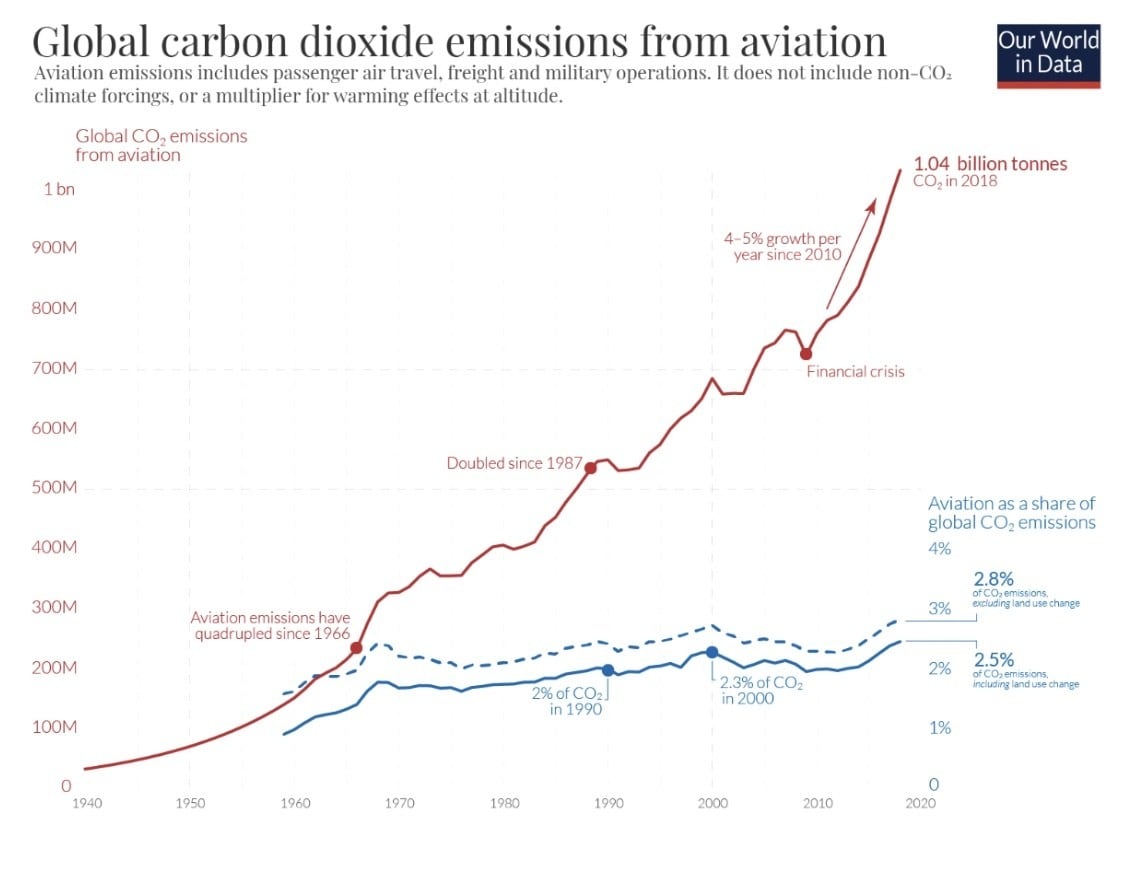
The aviation industry has adopted the goal of reaching net-zero carbon emissions by 2050. In October, the UN’s International Civil Aviation Organization (ICAO) led two weeks of negotiations involving 184 nations to agree on CO2 emissions reduction measures. These include ramping up innovative aircraft technologies, “streamlining” flight operations and the increased production and use of sustainable aviation fuels (SAF).
“States’ adoption of this new long-term goal for decarbonized air transport, following the similar commitments from industry groups, will contribute importantly to the green innovation and implementation momentum which must be accelerated over the coming decades to ultimately achieve emissions-free powered flight,” said the President of the ICAO Council, Salvatore Sciacchitano.
“As we move from commitment to action, it is essential for the industry to be backed by governments with a decarbonization goal,” said Laia Barbarà, the Industry Decarbonization Lead for Aviation at the World Economic Forum. “ICAO’s Long Term Aspirational Goal (LTAG) of net zero by mid-century is a great step forward in that direction. The World Economic Forum stands ready to support ICAO, governments and the private sector globally as they work on next steps,” she added.
Making aviation fuel more sustainable
Sustainable Aviation Fuels can reduce emissions by 80% according to the International Air Transport Association (IATA). SAF can be made from several sources ranging from agricultural waste to carbon captured from the air.
It is fully compatible with existing aircraft and fueling infrastructure. However, high production costs and limited supply has slowed its adoption. It is estimated that SAF comprises less than 0.1% of all jet fuel currently used.
IATA estimates that SAF could make up around 65% of the emissions reduction needed by aviation to reach net-zero by 2050. But it says “this will require a massive increase in production in order to meet demand. The largest acceleration is expected in the 2030s as policy support becomes global, SAF becomes competitive with fossil kerosene and credible offsets become scarcer.”
Have you read?
How to claim your flight emissions when flying on sustainable aviation fuels, fuelling sustainable aviation for the long haul, creating zero-emission aviation with hydrogen and electric power, offsetting emissions from aviation.
IATA says the aviation industry won’t be able to completely eliminate emissions at source, and will need to mitigate the rest using a variety of offsetting mechanisms. This is the process where emissions are compensated for by the financing of a reduction in emissions elsewhere.
ICAO adopted the Carbon Offsetting and Reduction Scheme for International Aviation (CORSIA) in 2016, which has been heralded as the first global market-based measure for any sector. IATA says “CORSIA aims to stabilize international civil aviation net CO2 emissions at 2019 levels, from 2021, using offsetting programs.” It adds, “we envisage that as new technology such as SAF becomes widespread, the need for offsets will diminish”.
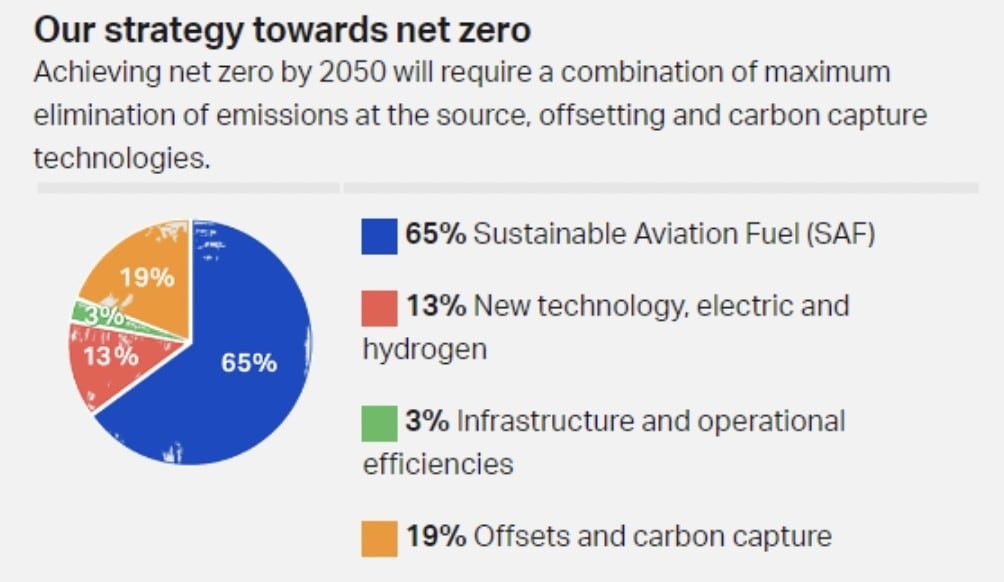
Electric and hydrogen can also help reduce aviation emissions
With short-haul flights of fewer than 600 miles accounting for more than 17% of airline emissions , new technologies like electric and hydrogen-powered aircraft are also being developed. It’s been estimated that all flights of fewer than 2,500 miles, which make up more than half of all CO2 emissions from aviation, could be electrified or powered by hydrogen.
European aviation giant Airbus is developing three types of hydrogen-fuelled zero-emission commercial aircraft which it says could enter service from 2035. They are: a turboprop plane carrying up to 100 passengers with a range of more than 1,000 nautical miles; a turbofan design (120-200 passengers) with a range of 2,000-plus nautical miles; and a “blended-wing body” design (up to 200 passengers) which could also fly over 2,000 nautical miles.
However, in order for hydrogen to be a carbon-neutral aviation fuel, it will need to be produced using sustainable methods. The ClimateWorks Foundation says “usage of green hydrogen (that is hydrogen produced using additional renewable energy) must be incentivized to achieve the deep decarbonization required from the aviation sector”.
Business and government leaders need to align on a meaningful pathway to accelerate aviation’s decarbonization for more companies to adopt SAF. The Clean Skies for Tomorrow Coalition is a global initiative to facilitate the transition to net-zero flying by 2050 - by accelerating the deployment of sustainable aviation fuel. Co-led by the World Economic Forum, the coalition of more than 100 companies is aiming to power global aviation with 10% SAF by 2030.
Emissions from mobility will increase through 2050, jeopardising our chances to combat climate change. By electrifying urban fleets, we can mitigate more than 70% of mobility CO2 urban emissions, remove 50% of city air pollution, and electrify rides to everyone - making the electrification transition more effective and equitable.
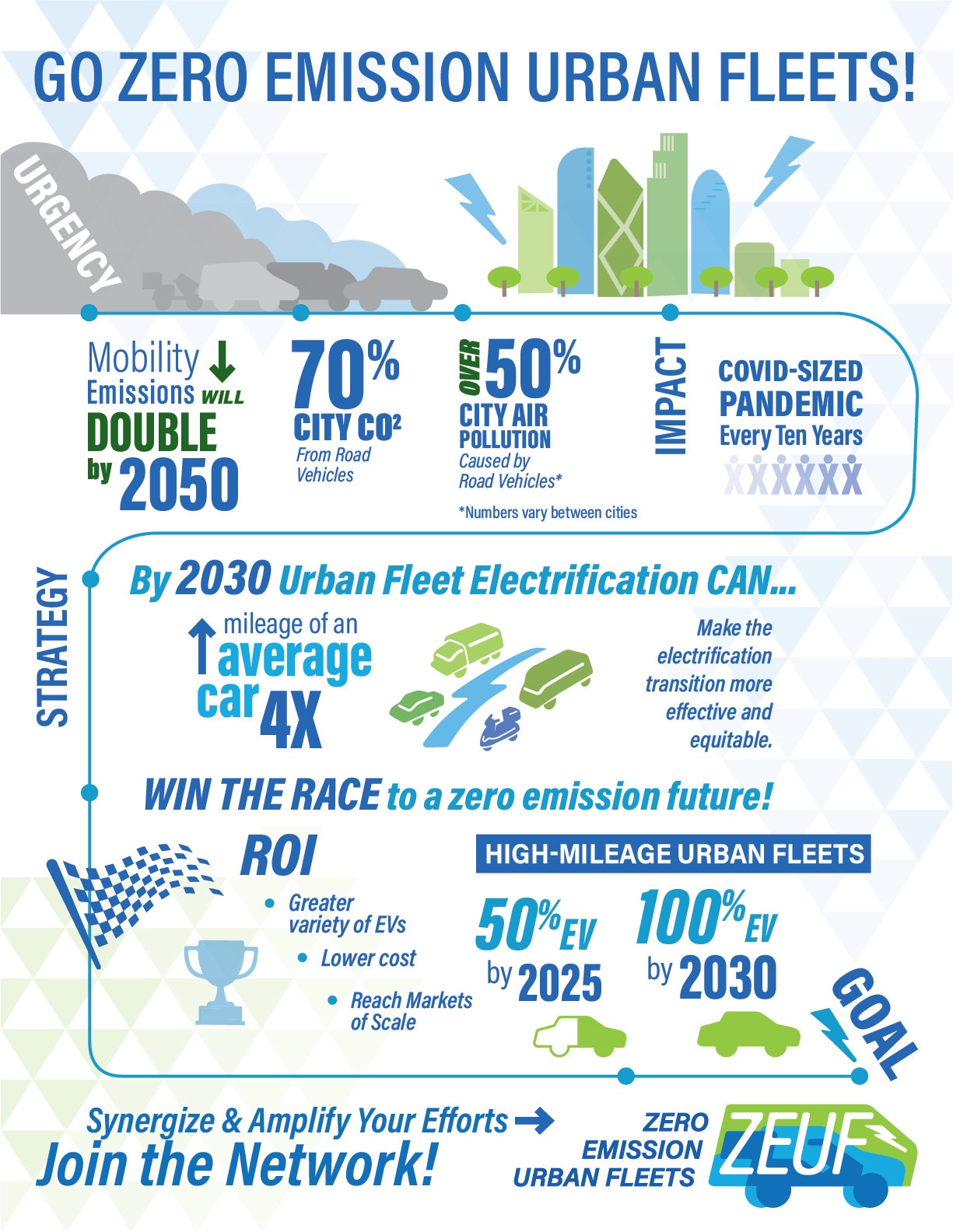
Understanding that coordination is needed to create tangible progress, this February the World Economic Forum launched a collaborative umbrella network that will help sync and synergize related global initiatives: the Zero Emissions Urban Fleets (ZEUF) network.
ZEUF is a network of stakeholders for accelerating urban fleets electrification, targeting 100% by 2030. Initiated by the World Economic Forum’s Global New Mobility Coalition in partnership with Uber, T&E, EuroCities, Free Now, LeasePlan, Door2Door, Lime, Blot, Voi, AEDIVE, Polis, The Climate Group, Race to Zero, and others, ZEUF is an open network that convenes periodically to facilitate informal know-how exchange and efforts coordination.
Don't miss any update on this topic
Create a free account and access your personalized content collection with our latest publications and analyses.
License and Republishing
World Economic Forum articles may be republished in accordance with the Creative Commons Attribution-NonCommercial-NoDerivatives 4.0 International Public License, and in accordance with our Terms of Use.
The views expressed in this article are those of the author alone and not the World Economic Forum.
Related topics:
The agenda .chakra .wef-n7bacu{margin-top:16px;margin-bottom:16px;line-height:1.388;font-weight:400;} weekly.
A weekly update of the most important issues driving the global agenda
.chakra .wef-1dtnjt5{display:-webkit-box;display:-webkit-flex;display:-ms-flexbox;display:flex;-webkit-align-items:center;-webkit-box-align:center;-ms-flex-align:center;align-items:center;-webkit-flex-wrap:wrap;-ms-flex-wrap:wrap;flex-wrap:wrap;} More on Climate Action .chakra .wef-nr1rr4{display:-webkit-inline-box;display:-webkit-inline-flex;display:-ms-inline-flexbox;display:inline-flex;white-space:normal;vertical-align:middle;text-transform:uppercase;font-size:0.75rem;border-radius:0.25rem;font-weight:700;-webkit-align-items:center;-webkit-box-align:center;-ms-flex-align:center;align-items:center;line-height:1.2;-webkit-letter-spacing:1.25px;-moz-letter-spacing:1.25px;-ms-letter-spacing:1.25px;letter-spacing:1.25px;background:none;padding:0px;color:#B3B3B3;-webkit-box-decoration-break:clone;box-decoration-break:clone;-webkit-box-decoration-break:clone;}@media screen and (min-width:37.5rem){.chakra .wef-nr1rr4{font-size:0.875rem;}}@media screen and (min-width:56.5rem){.chakra .wef-nr1rr4{font-size:1rem;}} See all
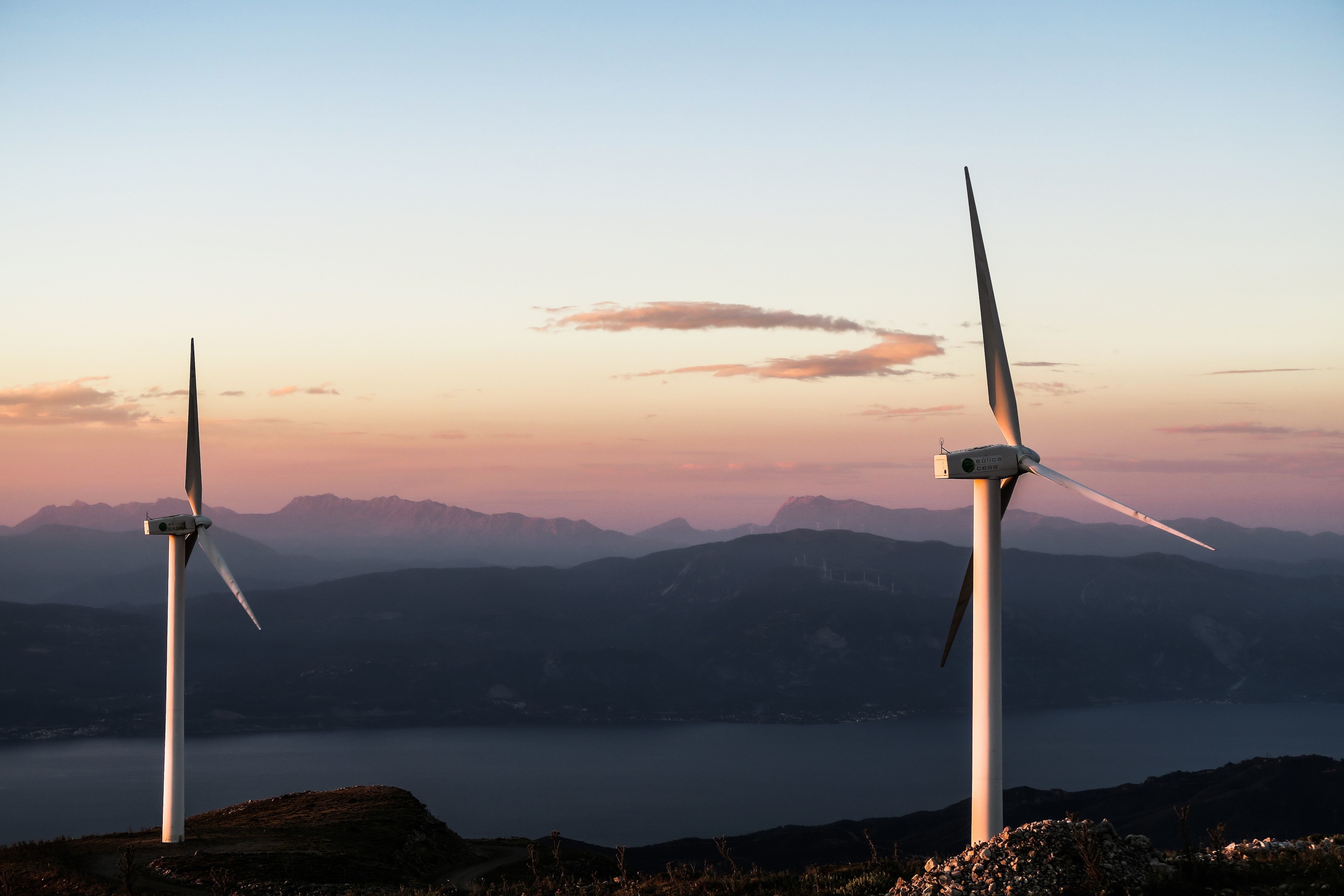
Climate finance: What are debt-for-nature swaps and how can they help countries?
Kate Whiting
April 26, 2024
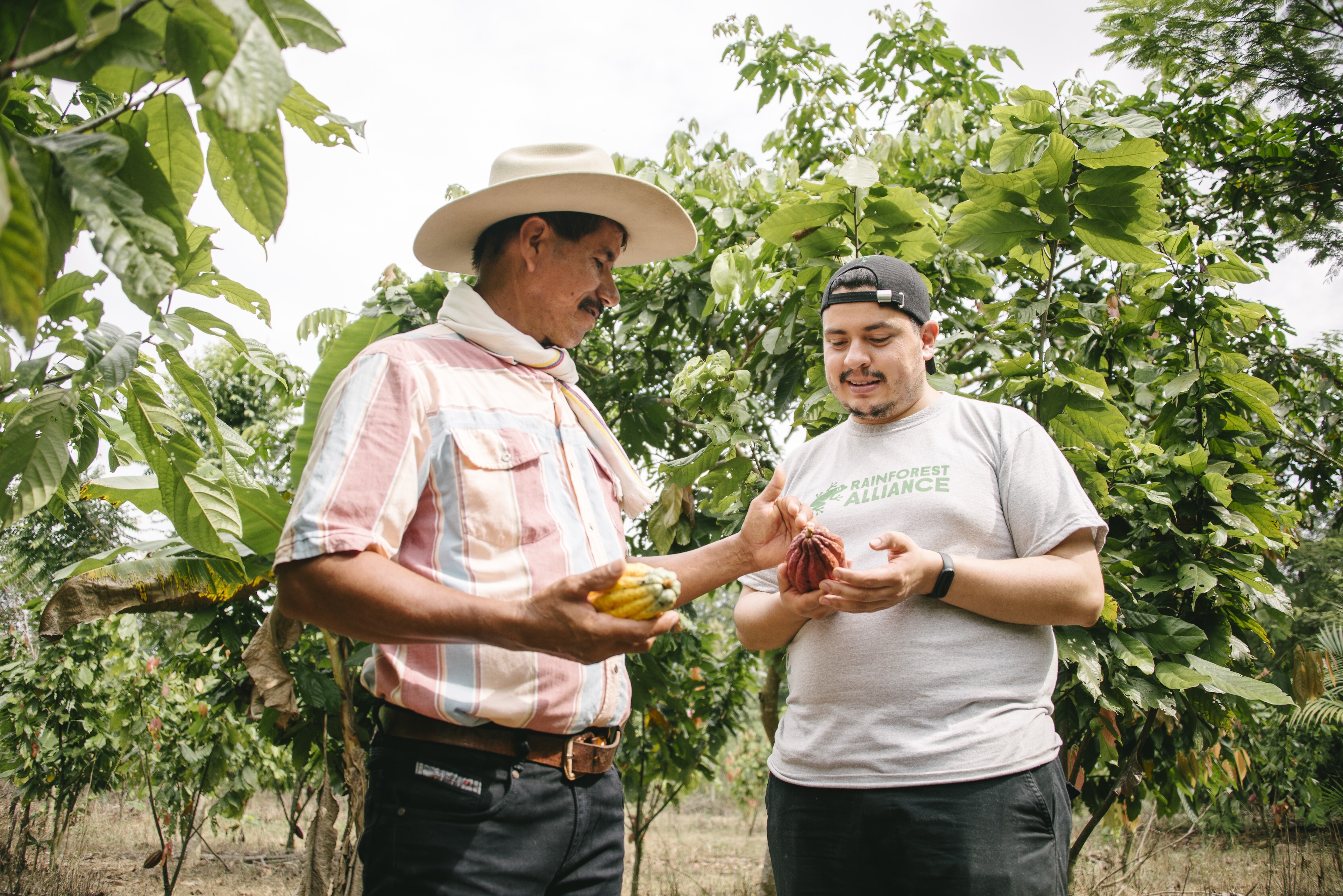
Beyond greenwashing: 5 key strategies for genuine sustainability in agriculture
Santiago Gowland
April 24, 2024
How Indigenous expertise is empowering climate action: A case study from Oceania
Amanda Young and Ginelle Greene-Dewasmes
April 23, 2024
What is desertification and why is it important to understand?
Andrea Willige
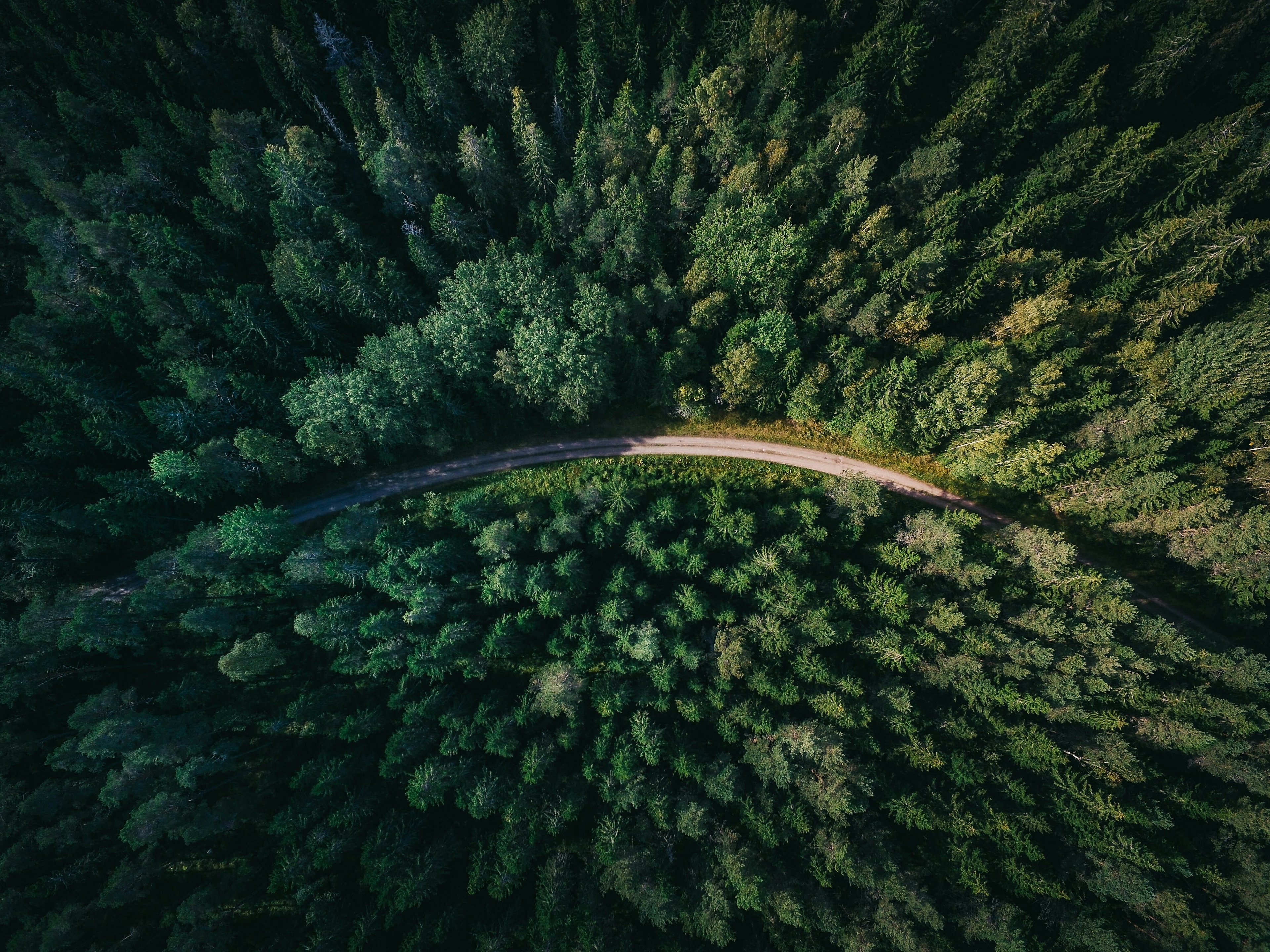
Global forest restoration goals can be achieved with youth-led ecopreneurship
Agustin Rosello, Anali Bustos, Fernando Morales de Rueda, Jennifer Hong and Paula Sarigumba
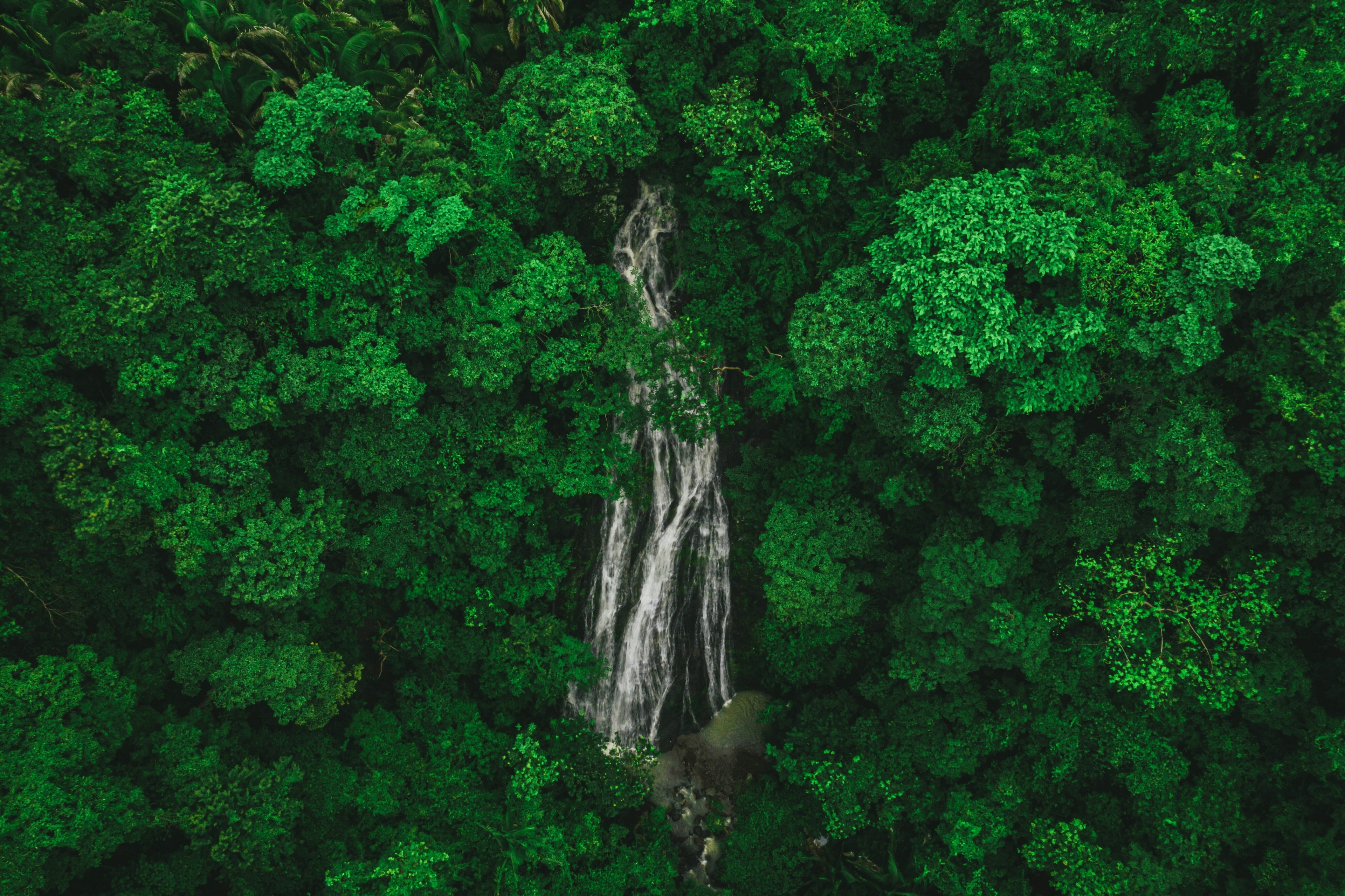
The planet’s outlook is in our hands. Which future will we incentivize?
Carlos Correa
April 22, 2024

ICAO Carbon Emissions Calculator (ICEC) //
ICAO has developed a methodology to calculate the carbon dioxide emissions from air travel for use in offset programmes. The methodology applies the best publicly available industry data to account for various factors such as aircraft types, route-specific data, passenger load factors and cargo carried.
The ICAO Carbon Emissions Calculator allows passengers to estimate the emissions attributed to their air travel. It is simple to use and requires only a limited amount of information from the user. ICEC is the only internationally approved tool to estimate carbon emissions from air travel.
Please contact us or refer FAQ or see the accompanying methodology to the ICAO Carbon Emissions Calculator for additional information.
- International edition
- Australia edition
- Europe edition
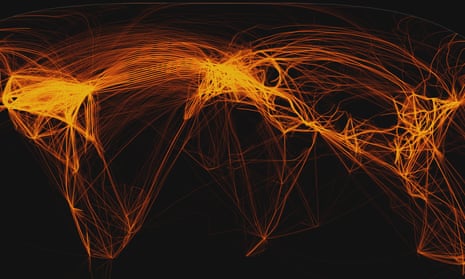
Carbon calculator: find out how much CO2 your flight will emit
The Guardian’s carbon calculator works out the emissions on flights from the world’s 100 busiest airports, as well as from selected UK airports
The aviation sector is one of the fastest-growing polluters. It currently accounts for about 2% of global emissions but that figure could more than double by 2050 according to research by Manchester Metropolitan University – or triple if planes don’t become substantially more fuel efficient. New airports continue to open and new routes are being added to airline schedules. According to forecasts, 2019 will be another record-breaking year for air travel, with passengers expected to fly a total of 8.1tn km, up 5% from last year.
What can you do as an individual? Avoid flying and opt for alternative modes wherever possible. If you do fly, you can work out the CO2 emissions generated by your flight using this calculator.
Read this article by John Vidal to find out about the growing number of carbon-offsetting schemes and how they work. Make your flight count – travel with an ethical holiday company and look for holidays that make a positive difference to the destination you’re visiting .
This article was corrected on 22 November 2019 to clarify the inputs used for the calculator.
- Air transport
Most viewed
- Work & Careers
- Life & Arts
Become an FT subscriber
Try unlimited access Only $1 for 4 weeks
Then $75 per month. Complete digital access to quality FT journalism on any device. Cancel anytime during your trial.
- Global news & analysis
- Expert opinion
- Special features
- FirstFT newsletter
- Videos & Podcasts
- Android & iOS app
- FT Edit app
- 10 gift articles per month
Explore more offers.
Standard digital.
- FT Digital Edition
Premium Digital
Print + premium digital, weekend print + standard digital, weekend print + premium digital.
Today's FT newspaper for easy reading on any device. This does not include ft.com or FT App access.
- 10 additional gift articles per month
- Global news & analysis
- Exclusive FT analysis
- Videos & Podcasts
- FT App on Android & iOS
- Everything in Standard Digital
- Premium newsletters
- Weekday Print Edition
- FT Weekend Print delivery
- Everything in Premium Digital
Essential digital access to quality FT journalism on any device. Pay a year upfront and save 20%.
- Everything in Print
Complete digital access to quality FT journalism with expert analysis from industry leaders. Pay a year upfront and save 20%.
Terms & Conditions apply
Explore our full range of subscriptions.
Why the ft.
See why over a million readers pay to read the Financial Times.
International Edition
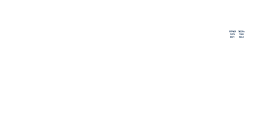
Cars, planes, trains: where do CO2 emissions from transport come from?
Transport accounts for around one-fifth of global co₂ emissions. three-quarters of this is from road transport..
Transport accounts for around one-fifth of global carbon dioxide (CO 2 ) emissions [24% if we only consider CO 2 emissions from energy] . 1
How do these emissions break down? Is it cars, trucks, planes or trains that dominate?
In the chart here we see global transport emissions in 2018. This data is sourced from the International Energy Agency (IEA) .
Road travel accounts for three-quarters of transport emissions. Most of this comes from passenger vehicles – cars and buses – which contribute 45.1%. The other 29.4% comes from trucks carrying freight.
Since the entire transport sector accounts for 21% of total emissions, and road transport accounts for three-quarters of transport emissions, road transport accounts for 15% of total CO 2 emissions.
Aviation – while it often gets the most attention in discussions on action against climate change – accounts for only 11.6% of transport emissions. It emits just under one billion tonnes of CO 2 each year – around 2.5% of total global emissions [we look at the role that air travel plays in climate change in more detail in another article ] . International shipping contributes a similar amount, at 10.6%.
Rail travel and freight emits very little – only 1% of transport emissions. Other transport – which is mainly the movement of materials such as water, oil, and gas via pipelines – is responsible for 2.2%.
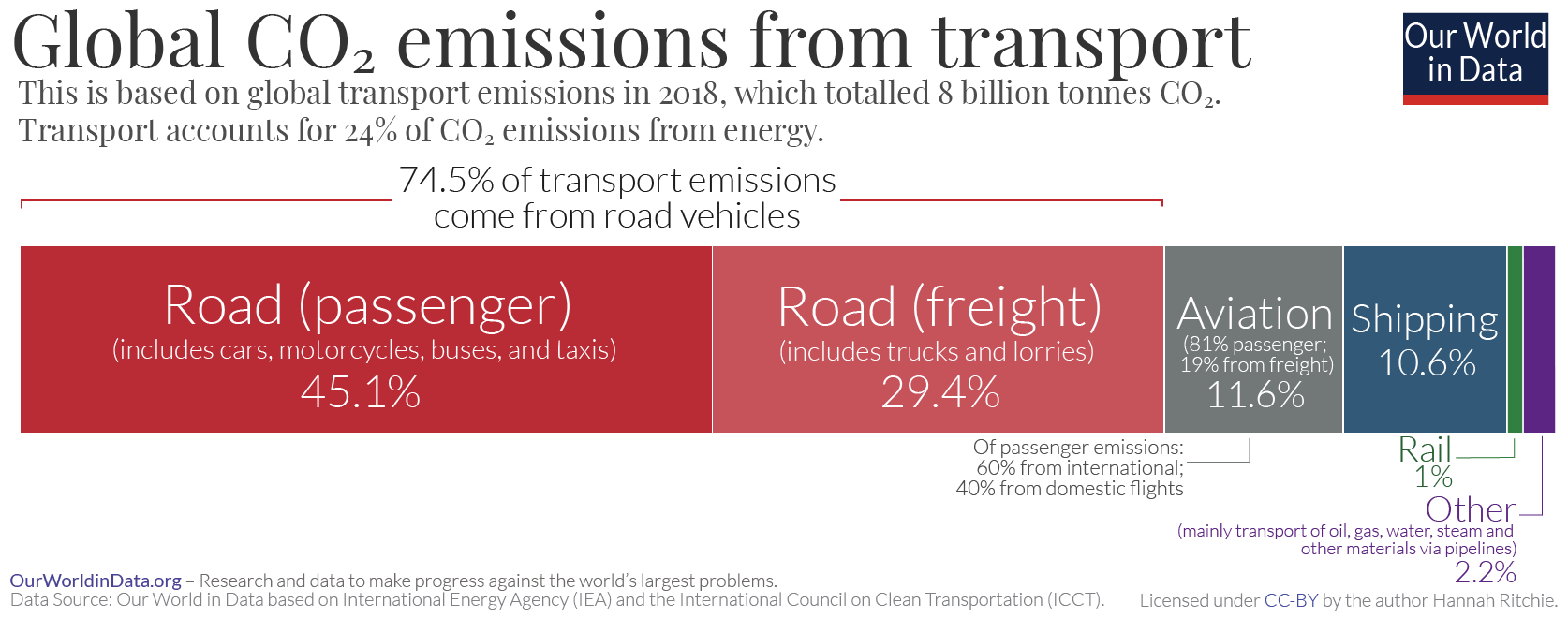
Towards zero-carbon transport: how can we expect the sector’s CO 2 emissions to change in the future?
Transport demand is expected to grow across the world in the coming decades as the global population increases, incomes rise, and more people can afford cars, trains, and flights. In its Energy Technology Perspectives report, the International Energy Agency (IEA) expects global transport (measured in passenger-kilometers) to double, car ownership rates to increase by 60%, and demand for passenger and freight aviation to triple by 2070. 2 Combined, these factors would result in a large increase in transport emissions.
But major technological innovations can help offset this rise in demand. As the world shifts towards lower-carbon electricity sources, the rise of electric vehicles offers a viable option to reduce emissions from passenger vehicles.
This is reflected in the IEA’s Energy Technology Perspective report. There it outlines its “Sustainable Development Scenario” for reaching net-zero CO2 emissions from global energy by 2070. The pathways for the different elements of the transport sector in this optimistic scenario are shown in the visualization.
We see that with electrification- and hydrogen- technologies some of these sub-sectors could decarbonize within decades. The IEA scenario assumes the phase-out of emissions from motorcycles by 2040; rail by 2050; and small trucks by 2060; and although emissions from cars and buses are not completely eliminated until 2070, it expects many regions, including the European Union; United States; China and Japan to have phased-out conventional vehicles as early as 2040.
Other transport sectors will be much more difficult to decarbonize.
In a paper published in Science , Steven Davis and colleagues looked at our options across sectors to reach a net-zero emissions energy system. 3 They highlighted long-distance road freight (large trucks), aviation, and shipping as particularly difficult to eliminate. The potential for hydrogen as a fuel, or battery electricity to run planes, ships, and large trucks is limited by the range and power required; the size and weight of batteries or hydrogen fuel tanks would be much larger and heavier than current combustion engines. 4
So, despite falling by three-quarters in the visualized scenario, emissions from these sub-sectors would still make transport the largest contributor to energy-related emissions in 2070. To reach net zero for the energy sector as a whole, these emissions would have to be offset by ‘negative emissions’ (e.g. the capture and storage of carbon from bioenergy or direct air capture ) from other parts of the energy system.
In the IEA’s net-zero scenario, nearly two-thirds of the emissions reductions come from technologies that are not yet commercially available. As the IEA states, “Reducing CO 2 emissions in the transport sector over the next half-century will be a formidable task.” 2
Global CO2 emissions from transport in the IEA's Sustainable Development Scenario to 2070 2
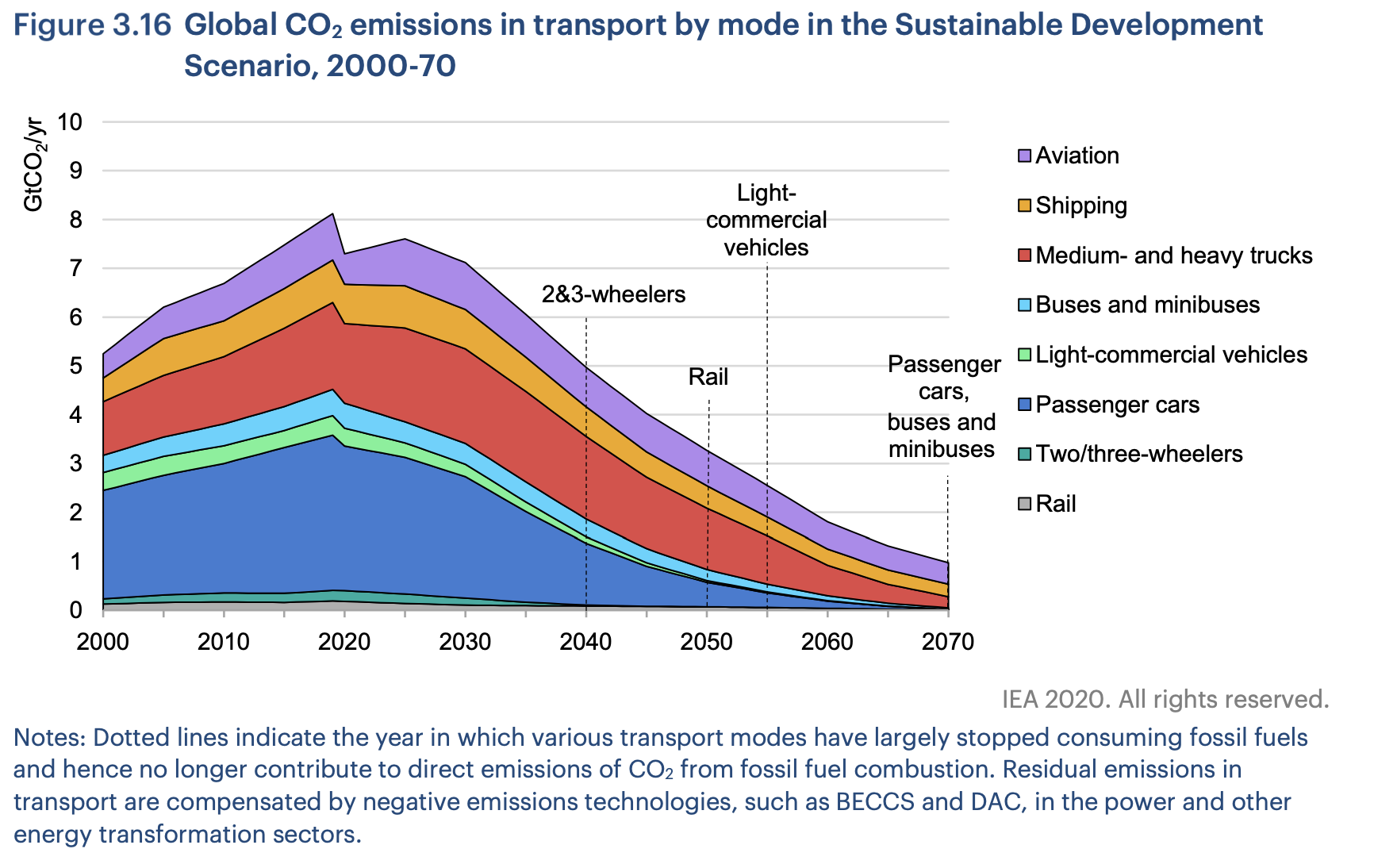
The World Resource Institute ’s Climate Data Explorer provides data from CAIT on the breakdown of emissions by sector. In 2016, global CO 2 emissions (including land use) were 36.7 billion tonnes of CO 2 ; emissions from transport were 7.9 billion tonnes of CO 2 . Transport therefore accounted for 7.9 / 36.7 = 21% of global emissions.
The IEA looks at CO 2 emissions from energy production alone – in 2018 it reported 33.5 billion tonnes of energy-related CO 2 [hence, transport accounted for 8 billion / 33.5 billion = 24% of energy-related emissions.
IEA (2020), Energy Technology Perspectives 2020 , IEA, Paris.
Davis, S. J., Lewis, N. S., Shaner, M., Aggarwal, S., Arent, D., Azevedo, I. L., ... & Clack, C. T. (2018). Net-zero emissions energy systems . Science , 360(6396).
Cecere, D., Giacomazzi, E., & Ingenito, A. (2014). A review on hydrogen industrial aerospace applications . International Journal of Hydrogen Energy , 39 (20), 10731-10747.
Fulton, L. M., Lynd, L. R., Körner, A., Greene, N., & Tonachel, L. R. (2015). The need for biofuels as part of a low carbon energy future . Biofuels, Bioproducts and Biorefining , 9(5), 476-483.
Cite this work
Our articles and data visualizations rely on work from many different people and organizations. When citing this article, please also cite the underlying data sources. This article can be cited as:
BibTeX citation
Reuse this work freely
All visualizations, data, and code produced by Our World in Data are completely open access under the Creative Commons BY license . You have the permission to use, distribute, and reproduce these in any medium, provided the source and authors are credited.
The data produced by third parties and made available by Our World in Data is subject to the license terms from the original third-party authors. We will always indicate the original source of the data in our documentation, so you should always check the license of any such third-party data before use and redistribution.
All of our charts can be embedded in any site.
Our World in Data is free and accessible for everyone.
Help us do this work by making a donation.

IMAGES
COMMENTS
In 2022 aviation accounted for 2% of global energy-related CO 2 emissions, having grown faster in recent decades than rail, road or shipping. As international travel demand recovers following the Covid-19 pandemic, aviation emissions in 2022 reached almost 800 Mt CO 2, about 80% of the pre-pandemic level.Many technical measures related to low-emission fuels, improvements in airframes and ...
Aviation accounts for 2.5% of global CO 2 emissions. I've calculated aviation's share of global emissions using the time series above and total CO 2 emissions data from the Global Carbon Project. 5. You can see the results in the chart below. In 2019, aviation accounted for 2.5% of CO 2 emissions from fossil sources and land use.
Over all, air travel accounts for about 2.5 percent of global carbon dioxide emissions — a far smaller share than emissions from passenger cars or power plants.
The COVID-19 pandemic halted global travel and reduced aviation by 45% in 2020, but CO 2 emissions persist for hundreds of years, so all emissions from all past flights are still at play. Recent ...
Air-travel climate-change emissions detailed for nearly 200 nations. Carbon emissions from flights that departed from low- and middle-income countries in 2019 totalled 417 million tonnes.
India and China, where air travel is surging, argued at the talks in Montreal this week that their air carriers would require until 2060 or 2070 to achieve net zero emissions. The 2050 target ...
CO₂ emissions by sector. CO₂ emissions embedded in trade. CO₂ emissions from aviation. CO₂ emissions from domestic air travel. CO₂ emissions from fossil fuels and land-use change Stacked area chart. CO₂ emissions from fossil fuels and land-use change Line chart. CO₂ emissions from international aviation.
New research that provides the most comprehensive calculations of aviation's impact on the climate finds that global air travel and transport is responsible for 3.5 percent of all drivers of climate change from human activities. ... 32.6 billion tonnes between 1940 and 2018, or roughly the total global CO 2 emissions for the year 2010.
Fly Net Zero is the commitment of airlines to achieve net zero carbon by 2050. At the 77th IATA Annual General Meeting in Boston, USA, on 4 October 2021, a resolution was passed by IATA member airlines committing them to achieving net-zero carbon emissions from their operations by 2050. This pledge brings air transport in line with supporting ...
A further study by Susanne Becek and Paresh Pant (2019) compared the contribution of each income group to global air travel emissions versus its share of world population. 2 This comparison is shown in the visualization. The 'richest' half of the world (high and upper-middle-income countries) were responsible for 90% of air travel emissions. 3
Due to the decline in air travel in response to COVID-19, there is a proposal to use only the 2019 emissions as the baseline. While CORSIA is an industry-level response, airlines individually are ...
Air travel has picked up this year following COVID-19 disruptions in 2020 and 2021. However, weekly seat capacity on commercial passenger airlines is still some way below 2019 levels. There are also regional differences in how airlines have recovered following the pandemic. As international travel was brought to an abrupt halt in 2020 at the ...
Although emissions from air travel dropped 40% in 2020 due to the COVID-19 pandemic, aviation demand is expected to recover and grow in the future 8, with emissions projected to reach as high as 1 ...
In 2022, global air travel emitted more than 780 Mt CO2, accounting for about 2% of global energy-related CO2 emissions that year. Emissions from aviation have steadily increased, quadrupling from ...
The global aviation industry has agreed to try to achieve net-zero emissions by 2050. The airline association IATA says using sustainable fuels and carbon offsetting will contribute more than 80% of the reduction in emissions. Other low-emission technologies like electric and hydrogen-powered aircraft are also being developed by major aviation ...
Yet, available data suggests that scope 3 emissions are significant (about 30% of scope 1 emissions). These findings have repercussions for the sector's net-zero ambitions, climate governance, consumer choices and air transport finance, as the overall contribution from air travel to climate change remains underestimated.
Aviation accounts for around 2.5% of global carbon dioxide (CO 2) emissions.But if you are someone who does fly, air travel will make up a much larger share of your personal carbon footprint. The fact that aviation is relatively small for global emissions as a whole, but of large importance for individuals that fly is due to large inequalities in the world.
The ICAO Carbon Emissions Calculator allows passengers to estimate the emissions attributed to their air travel. It is simple to use and requires only a limited amount of information from the user. ICEC is the only internationally approved tool to estimate carbon emissions from air travel. Please contact us or refer FAQ or see the accompanying ...
The Guardian's carbon calculator works out the emissions on flights from the world's 100 busiest airports, as well as from selected UK airports. The Guardian. Wed 31 Jul 2019 07.09 EDT. Last ...
In all, only 22 per cent of emissions from flights departing from Europe were caught by the regional carbon trading schemes in 2023, according to a report by Transport & Environment, an ...
Help us do this work by making a donation. The carbon footprint of travel is measured in grams of carbon dioxide-equivalents per passenger kilometer. This includes the impact of increased warming from aviation emissions at altitude.
Distance (each way): 2,583 miles or 4,156 km. Round-trip emissions per passenger: 1.5 metric tons CO2 equivalent. Avoiding this trip is as climate friendly as being vegetarian for: 2.8 years. Avoiding this trip is as climate friendly as carpooling for: 1.5 years. This many people in the world emit fewer greenhouse gases in one year: 802 million.
In spite of confusing public announcements, Russian President Vladimir Putin is well aware of the dangers of climate change. Last summer saw some of the most devastating fires in Siberia ever ...
The industry releases about four times as many planet-warming chemicals as the airline industry, according to the paper from scientists at the Lawrence Berkeley National Laboratory. Its emissions ...
It emits just under one billion tonnes of CO 2 each year - around 2.5% of total global emissions [we look at the role that air travel plays in climate change in more detail in another article]. International shipping contributes a similar amount, at 10.6%. Rail travel and freight emits very little - only 1% of transport emissions.