- Sustainable
- Energy Economy
- Energy Services


What is Round Trip Efficiency?
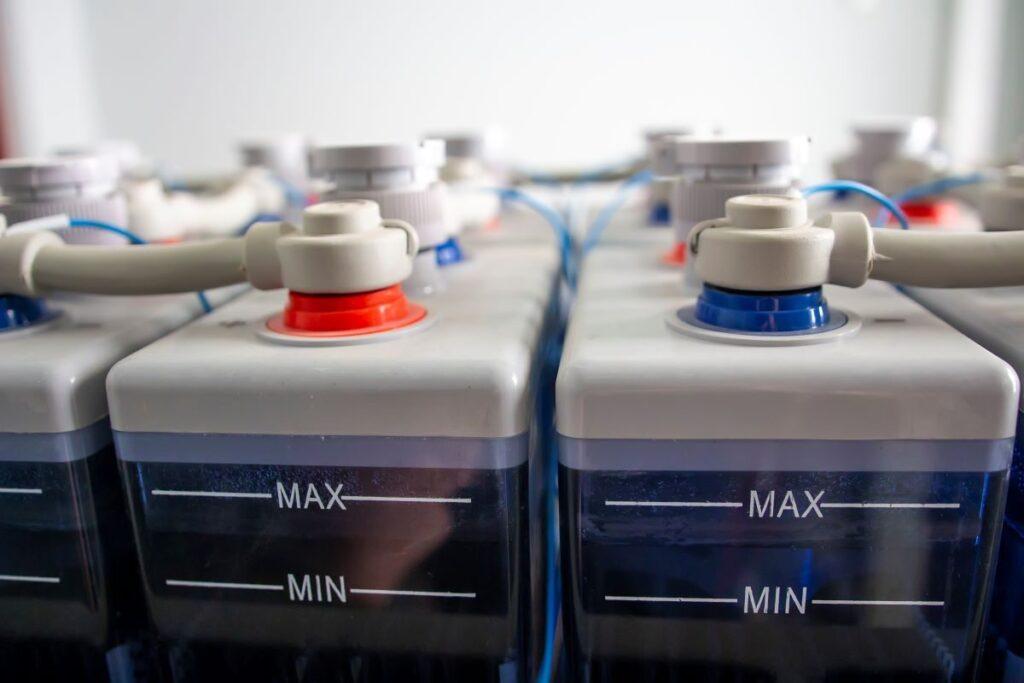
Energy storage systems function by taking in electricity, storing it, and subsequently returning it to the grid. The round trip efficiency (RTE), also known as AC/AC efficiency, refers to the ratio between the energy supplied to the storage system (measured in MWh) and the energy retrieved from it (also measured in MWh). This efficiency is expressed as a percentage (%).
The round trip efficiency is a crucial factor in determining the effectiveness of storage technology. A higher RTE indicates that there is less energy loss during the storage process, resulting in a more efficient overall system. Grid systems engineers strive for energy storage systems to achieve an 80% RTE whenever feasible, as it signifies a desirable level of efficiency and minimizes energy losses.
What Factors Can Affect the Round Trip Efficiency of an Energy Storage System?
The RTE of an energy storage system can be influenced by various factors, including:
1. Technology: Different storage technologies have varying round-trip efficiencies. For example, hydro storage typically ranges from 65% in older installations to 75-80% in modern deployments, while flywheels have efficiencies of about 80% to 90%. Some battery technologies can have round-trip efficiencies ranging from 75% to 90%.
2. Storage duration: Some technologies may experience leakage or energy loss over long-term storage, which can affect round-trip efficiency. It is important to consider the specific characteristics and limitations of the storage technology when evaluating its efficiency.
3. Age and condition of the system: Older storage systems may have lower round-trip efficiencies compared to newer ones. Factors such as wear and tear, component degradation, and maintenance practices can impact the overall efficiency of the system.
4. Charging and discharging rates: The speed at which energy is charged into and discharged from the storage system can affect its efficiency. Certain technologies may have lower efficiencies at high charging or discharging rates.
5. System design and control: The design and control strategies implemented in the energy storage system can influence its round-trip efficiency. Optimal system design, efficient power electronics, and effective control algorithms can improve the overall efficiency of the system.
6. Temperature: Temperature can have an impact on the performance and efficiency of energy storage systems. Extreme temperatures can affect the efficiency of certain storage technologies, such as batteries, leading to lower round-trip efficiencies.
Considering these factors is crucial when evaluating the round-trip efficiency of an energy storage system, as they can significantly affect its performance and effectiveness in storing and retrieving energy.
Must Read: What is Power Conversion Efficiency?
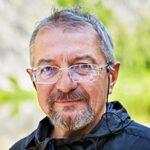
Elliot is a passionate environmentalist and blogger who has dedicated his life to spreading awareness about conservation, green energy, and renewable energy. With a background in environmental science, he has a deep understanding of the issues facing our planet and is committed to educating others on how they can make a difference.
Related Posts
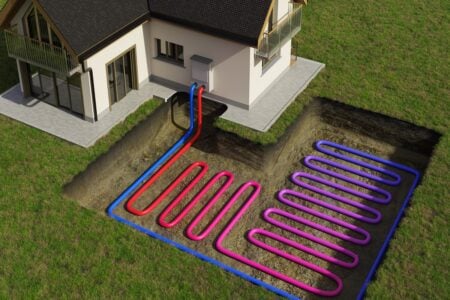
What is Heating Seasonal Performance Factor (HSPF)?
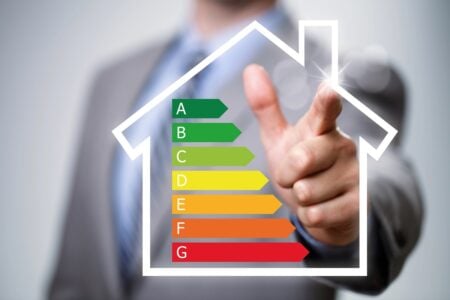
What is Annual Fuel Utilization Efficiency (AFUE)?
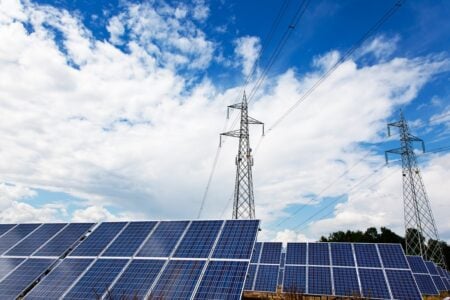
What is Grid Parity?
Save my name, email, and website in this browser for the next time I comment.
Type above and press Enter to search. Press Esc to cancel.
- POWER Plant ID
- POWER Events
- Connected Plant
- Distributed Energy
- International
- COVID-19 Coverage
- Carbon Capture
- Climate change
- Cybersecurity
- Distributed Power
- Electric Vehicles
- Energy Storage
- Environmental
- Instrumentation & Controls
- Legal & Regulatory
- Legislative
- Ocean/Marine
- Physical security
- Plant Design
- Power Demand
- Research and Development
- Supply Chains
- Tidal Power
- Waste to Energy
- About POWER
- Privacy Policy
- Diversity, Equity, Inclusion & Belonging
- Accessibility Statement
Don’t Neglect Round-Trip Efficiency and Cost of Charging When Considering Levelized Cost of Storage
The world is moving toward renewable sources for electricity generation in an attempt to reduce fossil-fuel reliance. But wind and solar can’t provide a consistent flow of power 24/7, and grid operators have realized that new electricity generation needs to be paired with storage to manage periods with no sun or wind.
The decreasing cost of lithium-ion batteries has made battery energy storage systems (BESS) more affordable; however, the cost of battery storage systems represents only 20%-25% of any project’s lifetime cost. Power equipment, land, site work, cabling, project design and management, grid integration, transportation, and other related up-front costs represent another 25%.
So, what makes up the other ~50%? Operations and maintenance, otherwise known as O&M, represent a few percentage points. O&M generally includes expenses associated with maintaining, repairing, and operating energy storage systems over their lifespan. The rest comes from the cost of electricity to charge the system, which is significantly affected by the system’s overall round-trip efficiency (RTE).
Why RTE and Cost of Energy Matter
Levelized cost of storage (LCOS) is a metric used to determine the cost per unit of energy discharged from an energy storage system. The calculation is usually expressed in dollars per megawatt hour (MWh) and includes initial costs plus operating costs divided by the energy discharged over the asset’s service life.
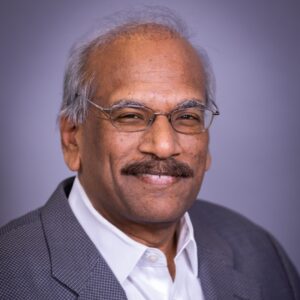
There are dozens of potential variables that may be used to determine the true levelized cost of storage, and different vendors will add, omit, or adjust different ones to put their products in the best light. This is why it’s so important to understand the role of RTE and cost of energy in a storage system, because they often have the biggest impact. These are also components that vendors with low-RTE technologies will most often discount (or omit altogether).
Round-trip efficiency is a measure of the amount of energy put into a system compared to the amount dispatched, and is expressed as a percentage. A system with a high RTE (75%+) is able to dispatch most of the energy fed into it. A low RTE indicates that the system loses a considerable amount of energy, often to heat arising from irreversible side reactions or high internal cell resistance. Many long-duration energy storage systems have RTEs below 50%, creating a significant amount of energy waste.
For example, lithium-ion batteries generally have RTEs of 90%+. In contrast, lead-acid batteries have lower RTEs of around 70%, meaning that approximately 30% of charge energy is lost. RTEs for flow batteries can range from 50%–75%, while metal-air batteries could have RTEs as low as 40%.
If the electricity used to charge low-RTE batteries was free, efficiency might not matter much. But electricity always comes with a cost. Some might argue that during periods where supply exceeds demand, renewables could be used to charge batteries when they would otherwise be curtailed. There’s a logic to that, but curtailment periods can’t always be predicted.
Even if you’re using electricity that would otherwise be curtailed, you have to assign a monetary value. If a turbine is spinning or a solar panel is generating electricity and a battery system is storing that electricity, every component in the system is subject to normal wear and tear plus maintenance and replacement protocols—all of which have costs associated. Factors at play include:
Technology lifespan and degradation rate. An energy storage system’s service life is determined by technology and cycles. All energy storage systems deteriorate over time, making them less efficient at storing and discharging energy. The same goes for generation sources. From solar to wind to flow batteries to lithium-ion, the more the components are used, the shorter the lifespan and the sooner the need for repair, replacement or augmentation.
Maintenance costs. Solar panels, wind turbines, battery systems, transmission lines, and power equipment all have to be maintained. The more they’re used, the more often components need to be serviced or replaced.
Long-Duration Doesn’t Always Mean Lower LCOS
The latest buzzy term in the energy space is “long-duration energy storage,” or LDES for short. While there’s no single definition of what the term means, the term has generally come to describe a non-lithium storage technology that can provide energy for anywhere from 8 to 160 hours at a lower installed cost per MW than lithium-ion batteries or a standard natural gas turbine.
LDES isn’t confined to battery storage; non-battery technologies include compressed air, latent heat, flywheels, and more. In fact, pumped hydro currently accounts for the vast majority of all LDES capacity in the US, and will likely remain in that position for an extended time. Battery technologies being positioned for LDES use include flow batteries, zinc-based chemistries, metal air, nickel hydrogen, and more.
These technologies all work well and are generally safer than lithium-ion batteries, but they come with trade-offs. Many have high up-front costs and must be amortized over 30–40-year periods to be cost competitive. Some have very low energy densities, requiring significant amounts of land for installations above a few megawatt hours. Some are rate-limited and can’t discharge as quickly as needed for specific applications. Some have very restricted siting requirements. And maybe most importantly, many have RTEs below 60%, with a few at 40% or lower.
So, what does this all mean? The race is on to build a better storage system, and with no universal standard for calculating LCOS, every vendor is using a model that plays to the strength of their own technology. If you’re investigating a new storage technology, be sure to ask a few questions when LCOS numbers come up, such as:
How many years are they calculating when it comes to system life? Lithium-ion batteries usually have to be augmented or replaced somewhere between 10 and 15 years of use; vendors with low densities or high installed costs may calculate over 30–40 years to lower their LCOS while factoring in two or more replacement cycles for lithium-ion.
What are they using for the cost of electricity to charge the system, and how does that compare with your actual costs? Even if you’re only planning to charge the system during periods you’d normally be curtailing renewables, remember that there’s still a cost to running those systems. A system with a low RTE may end up having a much higher LCOS even when you’re paying very little for electricity.
Are they including the cost of land in their calculations? If you’re installing a storage facility in a rural area where land is cheap, this may not matter so much. But if you need to place storage in or near a high-cost-of-living area, cost of land (and availability) could be one of your primary concerns and should definitely play a role in the LCOS calculation.
Are they including installation tax credits (ITCs) or production tax credits (PTCs) in their calculations? If so, be sure that the numbers are correct for your projects, and that the same are being applied to any other technologies you’re evaluating.
— Mukesh Chatter is the CEO of Alsym Energy , a technology company developing a low-cost, high-performance rechargeable battery chemistry that is free of lithium and cobalt.
SHARE this article

Today in Energy
- Recent articles
- liquid fuels
- natural gas
- electricity
- oil/petroleum
- production/supply
- consumption/demand
- exports/imports
- international
- forecasts/projections
- steo (short-term energy outlook)
Utility-scale batteries and pumped storage return about 80% of the electricity they store
Electric energy storage is becoming more important to the energy industry as the share of intermittent generating technologies, such as wind and solar, in the electricity mix increases. Electric energy storage helps to meet fluctuating demand, which is why it is often paired with intermittent sources. Storage technologies include batteries and pumped-storage hydropower , which capture energy and store it for later use. Storage metrics can help us understand the value of the technology. Round-trip efficiency is the percentage of electricity put into storage that is later retrieved. The higher the round-trip efficiency, the less energy is lost in the storage process. According to data from the U.S. Energy Information Administration (EIA), in 2019, the U.S. utility-scale battery fleet operated with an average monthly round-trip efficiency of 82%, and pumped-storage facilities operated with an average monthly round-trip efficiency of 79%.
EIA’s Power Plant Operations Report provides data on utility-scale energy storage, including the monthly electricity consumption and gross electric generation of energy storage assets, which can be used to calculate round-trip efficiency. The metrics reviewed here use the finalized data from the Power Plant Operations Report for 2019—the most recent year for which a full set of storage data is available.
Pumped-storage facilities are the largest energy storage resource in the United States. The facilities collectively account for 21.9 gigawatts (GW) of capacity and for 92% of the country’s total energy storage capacity as of November 2020.
In recent years, utility-scale battery capacity has grown rapidly as battery costs have decreased. As batteries have been increasingly paired with renewables , they have become the second-largest source of electricity storage. As of November 20, 2020, utility-scale battery capacity had 1.4 GW of operational capacity. Another 4.0 GW of battery capacity is scheduled to come online in 2021, according to EIA’s Preliminary Electric Generator Inventory .
Although battery storage has slightly higher round-trip efficiency than pumped storage, pumped-storage facilities typically operate at utilization factors that are currently twice as high as batteries. Increasing durations among battery applications could shift battery operations toward services that reward longer output periods. For example, in 2015, the weighted average battery duration was a little more than 46 minutes, but by 2019, weighted average battery durations had doubled to 1.5 hours. The role of batteries and their capability to provide high levels of round-trip efficiency may become more important as batteries continue to be deployed and as the intermittent renewables share of the electricity mix grows.
Tags: storage , electricity

- ORIENTATION
- Instructor Information
- RESS PROGRAM
- CREATING A PRESENTATION
- WRITING STYLE GUIDES
- Getting Help
10.2 Key Metrics and Definitions for Energy Storage

Key Metrics and Definitions for Energy Storage
There are a few key technical parameters that are used to characterize a specific storage technology or system. Those characteristics will determine compatibility of the storage with a proposed application and will also have impact on its economic feasibility. Let us go through some definitions.
Storage Capacity
Capacity essentially means how much energy maximum you can store in the system. For example, if a battery is fully charged, how many watt-hours are put in there? If the water reservoir in the pumped hydro storage system is filled to capacity, how many watt-hours can be generated by releasing that water? Those amounts are determined by storage capacity.
Understandably, the capacity of any storage will increase with the system size. The more battery stacks are installed, the more electric energy can be put in for storage. The larger the water reservoir, the greater energy turnaround becomes possible. The system size should be matched with the load and specific application.
Storage capacity is typically measured in units of energy: kilowatt-hours (kWh), megawatt-hours (MWh), or megajoules (MJ). You will typically see capacities specified for a particular facility with storage or as total installed capacities within an area or a country.
Sometimes you will see capacity of storage specified in units of power (watt and its multiples) and time (hours).
For example: 60 MW battery system with 4 hours of storage . What does it mean?
60 MW means that the system can generate electricity at the maximum power of 60 MW for 4 hours straight. That also means that the total amount of energy stored in the system is:
60 MW x 4 hours = 240 MWh
But it can also provide less power if needed. For example, if the load only requires 20 MW, the system can supply it for 12 hours. The total amount of stored energy is the same, but it is used more slowly:
20 MW x 12 hours = 240 MWh
So power and time ratings give us a little bit more information: we not only know how much energy is stored, but can also define at what maximum rate this energy can be potentially used.
Check Your Understanding Questions 1 & 2 (Multiple Choice)
Energy density.
Energy density is often used to compare different energy storage technologies. This parameter relates the storage capacity to the size or the mass of the system, essentially showing how much energy (Wh) can be stored per unit cell, unit mass (kg), or unit volume (liter) of the material or device.
For example, energy densities for different types of batteries are listed in the table below [IES, 2011]:
Of course, we are interested to store as much energy as possible while using as small and light device as possible for this purpose. From the table above we can conclude, for example, that a fully charged Lead-Acid battery will run out of charge much sooner than a fully charged Li-ion battery of the same mass/size.
Energy density is related to capacity and determines the duration of power generation. Also materials with higher energy density help make the power block more compact, which is useful in portable electronics and vehicle applications.
Just for comparison, the energy density of the pumped hydro storage is 0.2—2 Wh/kg, which is rather low and requires significant masses of water and large reservoir size to deliver utility scale power.
Check Your Understanding Question 3 (Multiple Choice)
Power density.
Power density (measured in W/kg or W/liter) indicates how quickly a particular storage system can release power. Storage devices with higher power density can power bigger loads and appliances without going oversize. Imagine an electric vehicle accelerating from 0 to 60 MPH – which takes a lot of power. If you look at the table below, you will see why Li-ion battery remains the technology of choice for powering electric vehicles, even though some other battery types exhibit similar energy densities.
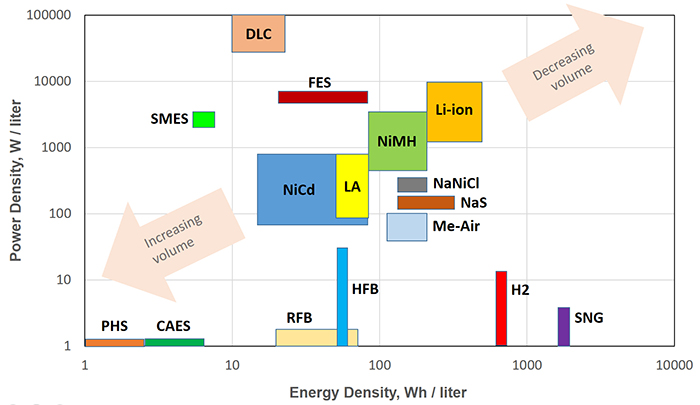
Key to abbreviations to Figure 10.2
CAES – Compressed Air Energy Storage DLS – Double Layer Capacitor FES – Flywheel Energy Storage H2 – Hydrogen storage LA – Lead Acid Battery Li-ion – Li ion Battery Me-air – Metal Air Battery NaNiCl – Sodium Nickel Chloride Battery NaS – Sodium Sulfur Battery NiCd – Nickel Cadmium Vented Battery NiMH – Nickel Metal Hydride Battery PHS – Pumped Hydro Storage RFB – Redox Flow Battery SMES – Superconducting Magnetic Energy Storage SNG – Synthetic Natural Gas
The technologies located in the lower left corner of the diagram (low energy density and low power density) take significant amount of space and material to enable the storage conversion and are mostly suitable for very large scale projects. Systems such as PHS and CAES also rely on the availability of specific landscape and geological features to accommodate the storage reservoirs.
The technologies located in the upper right corner of the diagram are most coveted for portable and efficient power supply, such as electric vehicles. These compact systems can carry a significant amount of energy and release it quickly on demand.
The technologies in the upper left corner are special devices that can be used in quick response electronics. These systems store small amounts of energy (and therefore charging can be fast), but are able to provide high power by releasing energy within short period of time.
Finally, the technologies in the lower right corner are characterized by slow charge and discharge, but the advantage is the total high amount of energy they are able to store, providing longer duration of energy supply.
Check Your Understanding Questions 4 & 5 (Multiple Choice)
Storage efficiency.
The main function of any storage device is to uptake and release power on demand. In case of a battery, for example, it would be electrochemical charge/discharge cycle; in case of pumped hydro storage, this process involves pumping water into the elevated reservoir and later releasing the flow through the turbine. Both charge and discharge processes include one or more energy conversions (Figure 10.3). In the figure, each arrow indicates the energy conversion from one form to another.
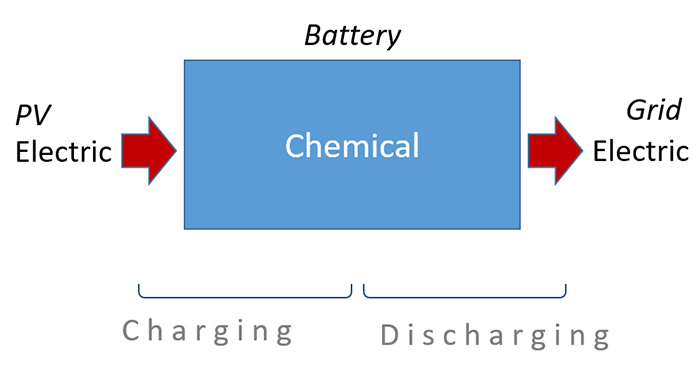
Regardless the number of transformations, the energy comes to its initial electric form, which is finally ready to be dispatched into the grid. This is the charge-discharge cycle, the "round trip".
In each conversion, energy is partially lost from the cycle and dissipated into the surroundings, and the efficiency of conversion at every step accounts for those losses.
Efficiencies of all energy conversion steps in this cycle are combined in the metric called round-trip efficiency , which essentially indicates the percentage of energy delivered by the storage system compared to the energy initially supplied to the storage system. The obvious goal is to minimize the conversion losses and thus maximize the overall storage efficiency.
Here are some round-trip efficiencies of various energy storage systems:
These numbers mean the following. For example, out of 1 MWh of energy spent to pump water up to the hydro storage, only 0.7-0.8 MWh will be available to use after the water is released to run the turbine and generator to produce electric power. The other 0.2-0.3 MWh of energy will be converted into non-useful forms of energy and “lost” from the cycle. Some of the energy losses occur in the auxiliary devices used in the energy storage process, very often in the form of waste heat. Furthermore, energy losses may be linked to the mechanical or material losses: for example, leaks and evaporation of water from pumped storage, air leaks in CAES, chemical degradation and incomplete reactions in batteries.
Check Your Understanding Questions 6 & 7 (Multiple Choice)
Round-Trip Energy Efficiency and Energy-Efficiency Fade Estimation for Battery Passport
Ieee account.
- Change Username/Password
- Update Address
Purchase Details
- Payment Options
- Order History
- View Purchased Documents
Profile Information
- Communications Preferences
- Profession and Education
- Technical Interests
- US & Canada: +1 800 678 4333
- Worldwide: +1 732 981 0060
- Contact & Support
- About IEEE Xplore
- Accessibility
- Terms of Use
- Nondiscrimination Policy
- Privacy & Opting Out of Cookies
A not-for-profit organization, IEEE is the world's largest technical professional organization dedicated to advancing technology for the benefit of humanity. © Copyright 2024 IEEE - All rights reserved. Use of this web site signifies your agreement to the terms and conditions.
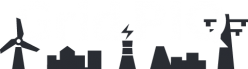
GridProjectIQ Documentation
Energy Storage System Efficiency
The round trip efficiency (RTE) of an energy storage system is defined as the ratio of the total energy output by the system to the total energy input to the system, as measured at the point of connection. The RTE varies widely for different storage technologies. A high value means that the incurred losses are low.
Reference Information
The typical RTE values for different technologies along with the source of information are provided below. If the reference provides a range of values instead of a single value, the median value of the range has been used.
- Lithium-ion (83%) : W. G. Manuel, “Energy Storage Study 2014”, 2014.
- Vanadium redox flow (75%) : V. Viswanathan, M. Kintner-Meyer, P. Balducci and C. Jin, “National Assessment of Energy Storage for Grid Balancing and Arbitrage, Phase II, Volume 2: Cost and Performance Characterization”, Sept. 2013.
- Sodium sulfur (75%) : W. G. Manuel, “Energy Storage Study 2014”, 2014.
- Advanced lead-acid (85%) : IEC, “Electrical Energy Storage: White Paper”, 2011.
- Flywheel (81%) : V. Viswanathan, M. Kintner-Meyer, P. Balducci and C. Jin, “National Assessment of Energy Storage for Grid Balancing and Arbitrage, Phase II, Volume 2: Cost and Performance Characterization”, Sept. 2013.
- Compressed air (50%) : IEC, “Electrical Energy Storage: White Paper”, 2011.
- Pumped hydro (81%) : V. Viswanathan, M. Kintner-Meyer, P. Balducci and C. Jin, “National Assessment of Energy Storage for Grid Balancing and Arbitrage, Phase II, Volume 2: Cost and Performance Characterization”, Sept. 2013.
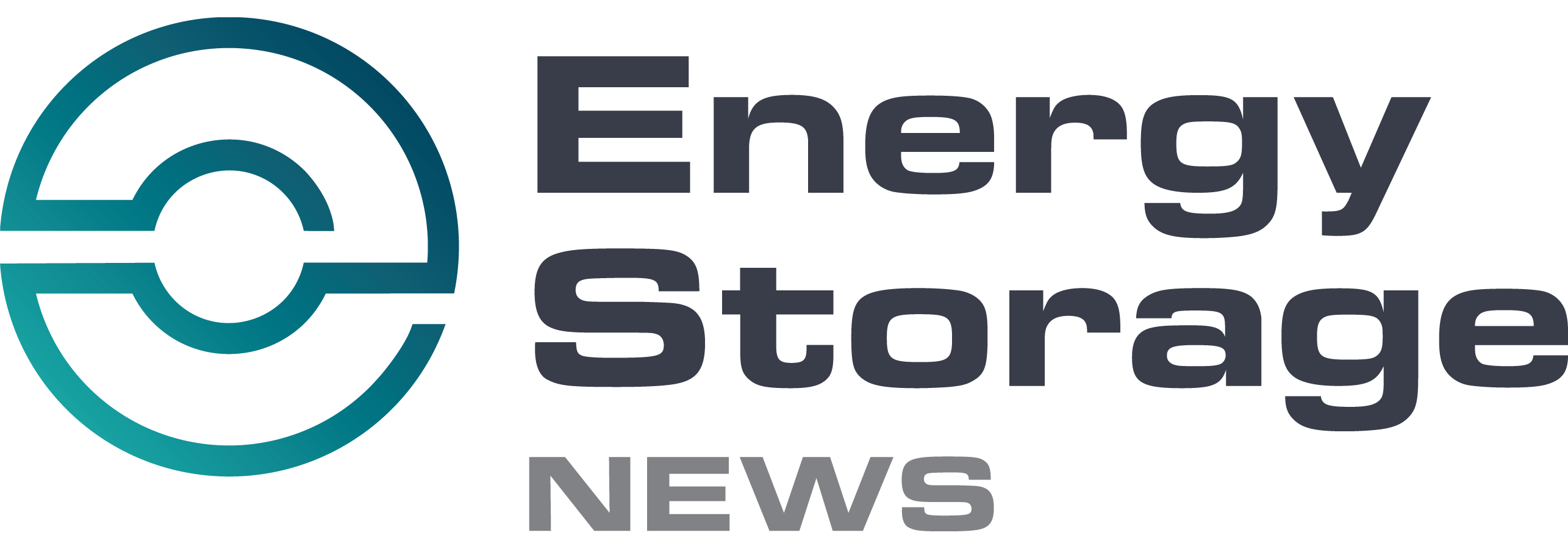
The Energy Storage Report 2024
Round-trip efficiency is key metric for assessing non-lithium energy storage alternatives, epcs say.
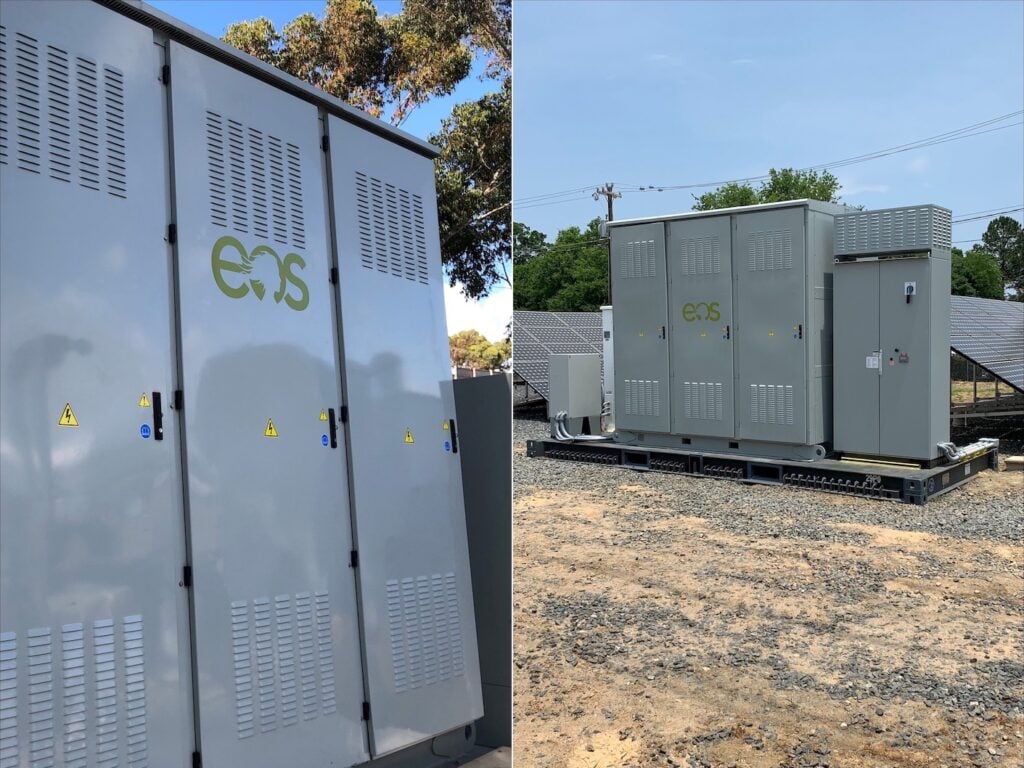
Round-trip efficiency of alternative storage technologies is the standout metric for assessing their potential versus lithium-ion, Energy-Storage.news has heard.
At last month’s RE+ national clean energy industry event, two US-based engineering, procurement and construction (EPC) companies offered their views after evaluating numerous non-lithium technologies.
Enjoy 12 months of exclusive analysis
- Regular insight and analysis of the industry’s biggest developments
- In-depth interviews with the industry’s leading figures
- Annual digital subscription to the PV Tech Power journal
- Discounts on Solar Media’s portfolio of events, in-person and virtual
Or continue reading this article for free
Their suitability for use in stationary energy storage system (ESS) applications hinges on a number of things, such as safety, abundance of materials and established supply chains.
Whereas in the past, there was perhaps a feeling that technology providers had increasingly sophisticated products and solutions without an immediately addressable market opportunity ahead of it, it appears that now the market is looking at non-lithium alternatives more seriously than before.
Factors include the desire to uncouple from existing supply chains: the ESS market still struggles to compete with high volume demand for batteries from the electric vehicle (EV) sector, meaning carmakers often absorb battery production long before their storage counterparts get a look in.
For the US in particular, it could also mean uncoupling from the global industry’s almost total dependency on Chinese manufacturers, materials suppliers and processors, which dominate the lithium battery space.
The US is supporting a domestic lithium battery value chain, but as we heard a while back from experts at three of the country’s network of National Laboratory facilities, it wouldn’t hurt to also explore the possibilities of creating value chains for next generation tech , aka ‘leapfrogging’ the current status quo.
There are many other reasons, such as the threat of thermal runaway. Although fires are exceedingly rare given the growth in deployment of lithium-ion, each fire that occurs increases the perception of risk. Many non-lithium alternatives, even electrochemical technologies like flow batteries, do not go into thermal runaway and pose even less fire risk than lithium batteries do.
Another big thing is that technologies besides lithium might actually be better suited to performing some of the applications stationary storage is increasingly being used for, particularly for those that require long durations of storage far in excess of the 4-hour or even 8-hour projects seen as the upper limits for cost-effective use of lithium battery energy storage system (BESS) technology.
Technology still ahead of market demand
However, the paramount consideration is whether they can store and release energy in an efficient enough way, or do it cheaply enough, to be a viable alternative, according to representatives of US EPC firms Kiewit and Burns & McDonnell.
In a presentation given at the RE+ exhibition’s Storage Central theatre space, Dr Helen Fischer from Kiewitt said that there are six different factors that affect the viability of energy storage technologies.
These factors are related to:
- Technology and performance
- Equipment and materials
- Overall economics
- Geographic requirements
- Whether some or all elements are based on existing, common industry experience
- How readily they can get regulatory backing.
In the first area of technology and performance, Fischer said round-trip efficiency is “the factor that matters when it comes to storage”.
Ben Echeverria, energy storage regulations and compliance expert at Burns & McDonnell, told Energy-Storage.news in an interview that he “absolutely” agreed with Fischer’s view on round-trip efficiency (RTE).
At present, a good RTE for non-lithium resources to even get into the conversation is being able to achieve “about 60% of what lithium can do”. Given that lithium technologies nowadays are routinely getting overall RTE in excess of 90%, versus about 75% for flow batteries, or roughly 80% for pumped hydro energy storage (PHES), it’s quite a steep curve.
That said, for technologies such as PHES, RTE may not matter as much as overall cost over the lifetime of the asset. Makers of newer storage technologies such as Form Energy, which has a 100-hour duration battery made using an iron-air chemistry, may also argue that while they may have RTE in the 70% – 80% range and relatively low energy density, their products can be made cheaply, using abundant raw materials which could play out much better across other metrics.
EPCs don’t tend to choose what types of technology go into projects they work on, it’s the customer’s choice. But the likes of Kiewitt and Burns & McDonnell will keep an eye on emerging technologies to have an understanding of where the market might be going next.
“The challenge that non-lithium really has is round-trip efficiency and the overall energy density,” Ben Echeverria said, while adding that those limitations could indeed be less of a dealbreaker if the technologies can scale.
At the same time, if the market demands them for being able to deliver large capacities of energy and long durations, essentially filling a gap where lithium cannot, or indeed, directly replacing the baseload role of thermal generation, that would also flip the equations on their head.
There is a whole industry ecosystem built around lithium and with costs going down with the scale of production and deployment, “it’s hard to go that route unless there’s a really significant financial use case for it,” Echeverria said.
“We see 2-hour [duration being suited] for a particular market or two, we see 4-hours being more of the generalised setup for the financial viability of energy storage [in the US]. So we’re still looking at this market financially, and, obviously, the power need or power requirement is a part of it, but in order to get someone to develop long-duration storage at scale, there has to be a financial feasibility to it,” Echeverria said.
The energy market doesn’t currently have a “significant need” for long-duration storage, not until large numbers of baseload power plants come offline, he said. The interest seen from the market hasn’t yet reached the same sort of commercial tipping point lithium has done in the last few years.
“Without that need, or without that immediate demand for it, then it just kind of sits over here as a somewhat of a pet project or pilot programme.”
Pilots and trial deployments are themselves no bad thing. Burns & McDonnell project manager Ben Kuisle noted that various utilities across the US are trying out LDES technologies, with Form Energy for example preparing to see its iron-air battery deployed by a number of them, most recently with Dominion Energy in Virginia .
“I think they’re looking at it now to be to be able to flip a switch when the time comes,” Kuisle said, while Kiewit’s Helen Fischer noted that the US government in its support for LDES has created a “commercial adoption readiness” scale to go alongside the more commonly used “technology adoption readiness” scale.
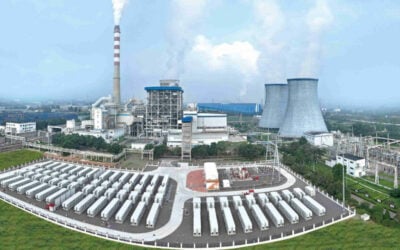
Energy storage market grew faster than ever in 2023, BESS was most invested-in energy tech, according to BNEF, IEA
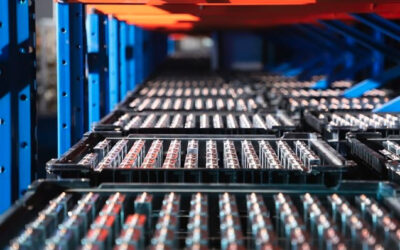
DNV Battery Scorecard ‘asks the questions every battery buyer should consider’
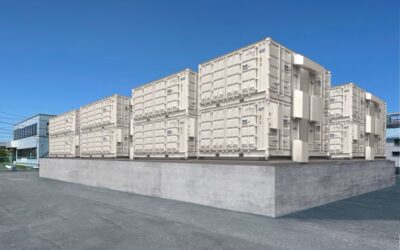
NGK supplying 230MWh sodium-sulfur battery storage for green hydrogen plant in Germany
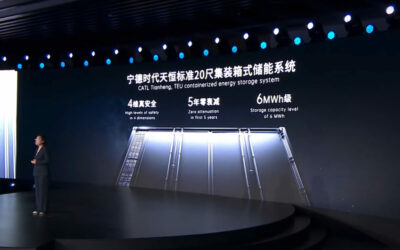
Has CATL cracked the battery ageing code? Industry reacts to ‘zero-degradation’ BESS claims
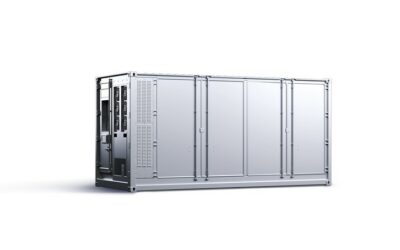
CATL unveils ‘five-year zero degradation’ BESS with 6.25MWh per container
Most popular, flexgen to deploy 15mw bess in puerto rico for arclight and infinigen, friday briefing: thermal plants offer brownfield opportunities but require ‘a lot of coordination’ , giga storage gets go-ahead for 2.4gwh belgium project, still choosing bess provider, caes developer corre energy hires rothschild for investment process, email newsletter.
- Advertising
- Terms of Use
- Terms of Sale
- Privacy Policy
- Cookie Policy
Thank you for visiting nature.com. You are using a browser version with limited support for CSS. To obtain the best experience, we recommend you use a more up to date browser (or turn off compatibility mode in Internet Explorer). In the meantime, to ensure continued support, we are displaying the site without styles and JavaScript.
- View all journals
- My Account Login
- Explore content
- About the journal
- Publish with us
- Sign up for alerts
- Open access
- Published: 19 April 2022
Reversible Power-to-Gas systems for energy conversion and storage
- Gunther Glenk ORCID: orcid.org/0000-0003-2540-838X 1 &
- Stefan Reichelstein ORCID: orcid.org/0000-0003-0989-6715 2
Nature Communications volume 13 , Article number: 2010 ( 2022 ) Cite this article
10k Accesses
41 Citations
146 Altmetric
Metrics details
- Energy economics
In the transition to decarbonized energy systems, Power-to-Gas (PtG) processes have the potential to connect the existing markets for electricity and hydrogen. Specifically, reversible PtG systems can convert electricity to hydrogen at times of ample power supply, yet they can also operate in the reverse direction to deliver electricity during times when power is relatively scarce. Here we develop a model for determining when reversible PtG systems are economically viable. We apply the model to the current market environment in both Germany and Texas and find that the reversibility feature of unitized regenerative fuel cells (solid oxide) makes them already cost-competitive at current hydrogen prices, provided the fluctuations in electricity prices are as pronounced as currently observed in Texas. We further project that, due to their inherent flexibility, reversible PtG systems would remain economically viable at substantially lower hydrogen prices in the future, provided recent technological trends continue over the coming decade.
Similar content being viewed by others
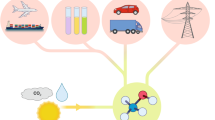

Solar methanol energy storage
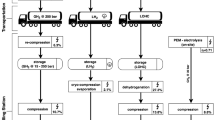
Optimal supply chains and power sector benefits of green hydrogen
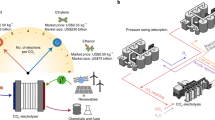
Techno-economic assessment of low-temperature carbon dioxide electrolysis
Introduction.
The large-scale deployment of intermittent renewable energy sources, like wind and solar, poses a growing challenge in terms of balancing energy demand and supply in real time 1 , 2 . Aside from storage in batteries 3 , 4 , electrolytic hydrogen production via Power-to-Gas (PtG) processes can absorb electricity during times of ample power supply and thereby yield hydrogen for industrial customers 5 , 6 , 7 . Conversely, PtG systems that are also capable of operating in the reverse direction can convert hydrogen back to electricity during periods of limited power supply and correspondingly high power prices 8 , 9 . Thus, reversible PtG systems can effectively connect the markets for hydrogen and electricity 10 , 11 , 12 and, in the process, limit the growing price volatility in electricity markets 13 , 14 .
Reversible PtG systems can be designed in a modular manner, for instance, by combining a one-directional electrolyzer for hydrogen production with a one-directional fuel cell or gas turbine for power generation 15 , 16 . While electrolyzers have been found to become increasingly cost-competitive in producing hydrogen 17 , 18 , fuel cells and gas turbines have so far been regarded as too expensive for converting hydrogen back to electricity that would subsequently be sold in wholesale markets 9 , 19 , 20 . In contrast, unitized regenerative fuel cells, which we refer to as integrated PtG systems, utilize the same equipment to deliver either hydrogen or electricity depending on the prevailing electricity prices at different points in time 21 , 22 , 23 .
This paper first develops an analytical model of the unit economics of reversible PtG systems. Our findings show that the technological characteristics of both modular and integrated systems entail certain ranges for hydrogen prices at which reversible PtG systems become cost-competitive. While modular systems require sufficiently low hydrogen prices in order for the reversibility feature to be valuable, integrated systems can be economically viable for higher hydrogen prices by primarily generating hydrogen but also providing electricity during times of limited power supply. Such operations will therefore not only increase the supply of hydrogen but also provide an effective buffer against the intermittency of renewable power sources.
The empirical part of our analysis calibrates the model in the context of the electricity markets in Germany and Texas. Despite improvements in the cost and conversion efficiency of modular PtG systems 24 , 25 , we confirm the findings of earlier studies that there is no economic case, either now or in the foreseeable future, for investing in modular systems that convert hydrogen back to electricity. In contrast, integrated PtG systems based on solid oxide cell (SOC) technology are shown to be competitive at current hydrogen prices, given sufficient variation in daily electricity prices, as is already encountered in the Texas market. For such systems, it is indeed efficient to mostly produce hydrogen and respond to sufficiently high electricity prices with electric power production. Owing to their relatively high capacity utilization, integrated systems are also positioned more competitively than one-directional electrolyzers on their own.
Finally, we project that if recent trends regarding the acquisition cost and conversion efficiency of solid oxide fuel cells continue, such reversible PtG systems will remain economically viable even in the presence of substantially lower hydrogen prices in the future. This is because the inherent flexibility in these systems enables them to respond to lower hydrogen prices by operating more frequently in reverse mode, delivering additional electricity to the power markets.
Real-time operation of reversible Power-to-Gas
We examine reversible PtG systems that can (i) produce hydrogen via water electrolysis and (ii) produce electricity from hydrogen and oxygen 26 . We refer to such systems as modular if the two production processes run on separate equipment, such as a one-directional electrolyzer for hydrogen production and a one-directional fuel cell or gas turbine for the reverse operation. In contrast, we refer to a unitized regenerative fuel cell based on, for instance, a SOC 10 , 27 or a proton exchange membrane (PEM) 22 , 28 technology as an integrated reversible PtG system. Such systems can carry out both production processes on the same equipment, yet they can only run in at most one direction at any point in time.
Since our interest is in the economics of reversible PtG systems, we focus on such systems operating on their own as price takers in a wholesale market for electricity in which prices are determined hourly based on supply and demand. Time is modeled as a continuous variable t ranging from 0 to 8760 h per year. Let q ( t ) denote the market price for electricity per kilowatt-hour (kWh) at time t . We initially assume that the annual distribution of power prices remains constant across the lifetime of the system. Symbols and acronyms are listed in Supplementary Table 1 .
Our model framework considers reversible PtG systems with a peak capacity in kilowatt (kW) of electricity input or output. The assumed size of a PtG system is in line with average capacity sizes reported in the literature 29 . PtG systems generally exhibit economies of scale over a certain range in the sense that system prices per kW, that is, the upfront capital cost decline as the capacity size increases up to a particular level 30 . The numerical calibration of our model relies on parameters for both system prices and operating costs that reflect average system sizes as reported in the existing literature 29 .
The basic version of our model makes the simplifying assumption that either reversible PtG system can be brought instantaneously from a cold start to full operating temperature without any loss in conversion efficiencies. Earlier work, however, shows that the process of heating SOCs up to operating temperature can require up to 20 min to prevent excessive material stress 31 . The electrical energy required for that heating amounts to a fraction of the energy needed for the subsequent electrolytic hydrogen production 32 . Reversible PtG systems based on PEM technology can be heated to operating temperature in less than 10 min 30 .
We examine the losses incurred by bringing either reversible PtG system from a cold start to full operating temperature in an extension to the basic model provided in Supplementary Note 1 . The extension explicitly accounts for (i) the time required to heat either reversible PtG system from a cold start to regular operating temperature, (ii) the energy required for the heating process, (iii) the cost of electricity or hydrogen incurred during the heating period, and (iv) the frequency of those heating periods in each year of operation. On the basis of conservative assumptions for all four of these frictions, our numerical results show that heating costs have only a small impact on the cost of either PtG system. The main reason is that the optimized PtG systems go only through a few heat-up phases per year.
Once the electrolyzer and fuel cell technologies we consider have reached their operating temperature, up- and down-ramping can be conducted in seconds 10 , 22 , 30 . The corresponding capacity factors reflect the percentage of the available capacity utilized at time t and can then be chosen flexibly on the interval [0, 1]. Efficiency losses incurred for maintaining the operating temperature are included in the conversion efficiencies considered throughout our analysis. Heat management is commonly more complex for high-temperature electrolyzers and fuel cells, such as SOC facilities, than for low-temperature PEM systems. Nevertheless, the cost of maintaining the operating temperature of well-insulated SOC systems is likely minor 32 .
If the modular system generates hydrogen at time t , it earns a “conversion price” consisting of the market price of hydrogen, p , per kilogram (kg) multiplied with the conversion rate of going from electricity to hydrogen (in kg/kWh). The hydrogen price, p , is modeled as time-invariant, because buyers and suppliers typically agree on time-invariant prices 33 . The corresponding conversion parameter \({\eta }_{h}^{o}(C{F}_{h}^{o})\) represents the amount of hydrogen (in kg) that can be generated from 1 kWh of electricity, given the capacity factor of \(C{F}_{h}^{o}\) at time t , with \(0\le C{F}_{h}^{o}\le 1\) . The variable cost of hydrogen generation equals q ( t ) plus a cost increment \({w}_{h}^{o}\) per kWh that accounts for consumable inputs, like water and reactants for deionizing the water, as well as any purchasing markups on the wholesale price of electricity.
Given a hydrogen price, p , the contribution margin per kWh from hydrogen production with the modular reversible PtG system at time t thus is:
with \(C{F}_{h}^{o}\) to be chosen at each point in time t .
Conversely, if the modular system generates electricity, it earns q ( t ) and incurs a variable cost that comprises p and an incremental cost, \({w}_{e}^{o}\) , per kWh of electricity for transporting hydrogen to the Gas-to-Power (GtP) system. To account for efficiency losses, the cost of hydrogen, p , is marked-up by the conversion rate for power generation, \({\eta }_{e}^{o}(C{F}_{e}^{o})\) (in kWh/kg). The shape of the functions \({\eta }_{h}^{o}(\cdot )\) and \({\eta }_{e}^{o}(\cdot )\) depends on the particular technology considered. The contribution margin of electricity generation per kWh at time t then becomes:
Efficient utilization of the existing capacity is obtained if the capacity factors are at each point in time chosen to maximize the total available contribution margin. While the modular system can run at full capacity in both directions, the 1st Law of Thermodynamics stipulates that the overall round-trip efficiency must satisfy the inequality \({\eta }_{h}^{o}(\cdot )\cdot {\eta }_{e}^{o}(\cdot )\le 1\) for all \(0\le C{F}_{h}^{o},C{F}_{e}^{o}\le 1\) . Consequently, at most one of the terms \([{\eta }_{h}^{o}(C{F}_{h}^{o})\cdot p-q(t)-{w}_{h}^{o}]\) or \(\left[q(t)-\frac{p}{{\eta }_{e}^{o}(C{F}_{e}^{o})}-{w}_{e}^{o}\right]\) will be positive for any given values \({w}_{h}^{o},{w}_{e}^{o}\ge 0\) . As illustrated in Fig. 1 (see Methods for formal derivations), efficient system utilization thus implies that the capacity factors be chosen so that \(C{F}_{h}^{o}\cdot C{F}_{e}^{o}=0\) . Specifically, the optimal capacity factors, \(C{F}_{h}^{o* }(t| p)\) and \(C{F}_{e}^{o* }(t| p)\) maximize pointwise the sum of the contribution margins in ( 1 ) and ( 2 )(see Methods for details). When aggregated across the hours of a year, the maximized contribution margins will be denoted by \(C{M}_{h}^{o}(p)\) and \(C{M}_{e}^{o}(p)\) .
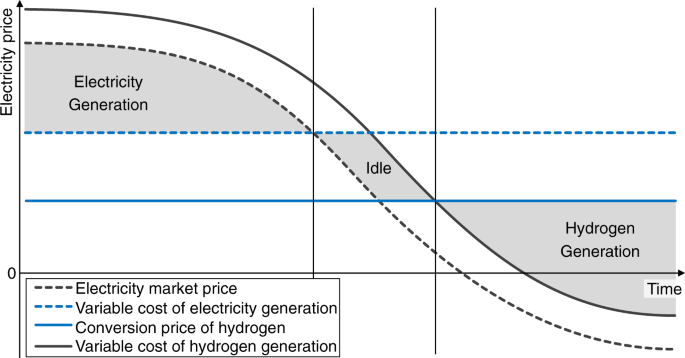
The figure illustrates the three alternative operating modes for either a modular or an integrated reversible PtG system that emerge for varying electricity prices. Wholesale electricity prices can turn negative as a result of surplus energy being supplied to the grid at certain hours.
For the integrated system, the economic trade-off is principally the same, except that the incremental cost and conversion rates may differ and instead assume the values w h , w e , η h ( ⋅ ), and η e ( ⋅ ), respectively. Once they are at operating temperature, unitized regenerative fuel cells based on SOC or PEM technology can rapidly switch between hydrogen and electricity production at full capacity 22 , 27 . Figure 1 illustrated that provided there are no sudden jumps in electricity prices, time intervals where electricity generation is valuable will typically be followed by a time interval in which the system is idle before entering a stretch of time where the regenerative fuel cell again becomes active in either mode of operation.
By construction, the integrated system faces the technical rather than economic “complementary slackness” condition C F h ⋅ C F e = 0 for all t . The corresponding contribution margins are:
for hydrogen production, and
for electricity. The capacity factors that maximize the sum of the contribution margins in ( 3 ) and ( 4 ), subject to the complementary slackness constraint, are denoted by \(C{F}_{h}^{* }(t| p)\) and \(C{F}_{e}^{* }(t| p)\) , respectively. Given these capacity factors, we denote by C M ( p ) the optimized aggregate contribution margin which is obtained as the total contribution margin obtained after integrating ( 3 ) and ( 4 ) across the hours of the year.
Cost competitiveness and the value of reversibility
A reversible PtG system is said to be cost-competitive if the required upfront investment in equipment yields a positive net present value in terms of discounted future cash flows. The discounted annual stream of optimized contribution margin of the system must then at least cover the initial equipment expenditure. For direct comparison, it will be convenient to capture this economic trade-off on a levelized basis. Analogous to the commonly known levelized cost of electricity, the levelized fixed cost (LFC) of a reversible PtG system reflects the unit acquisition cost of the system per kWh, including applicable fixed operating costs, corporate income taxes, and the cost of debt and equity 34 , 35 .
For the modular system, the LFC for the electrolyzer is denoted by \(LF{C}_{h}^{o}\) . As shown in Methods, the PtG subsystem is cost-competitive (positive net present value) if and only if at the prevailing market price for hydrogen, p :
Since the contribution margin from hydrogen is increasing in the selling price of hydrogen, there exists a unique break-even price, \({p}_{h}^{o}\) , such that PtG will be cost-competitive whenever \(p\ge {p}_{h}^{o}\) . Similarly, the Gas-to-Power subsystem is cost-competitive whenever:
with \(LF{C}_{e}^{o}\) denoting the corresponding LFC per kWh. Since the contribution margin from producing electricity is decreasing in the input price for hydrogen, p , there also exists a unique break-even price, \({p}_{e}^{o}\) , below which GtP will be cost-competitive.
By design, investors in a modular system retain the option of acquiring only one of the two subsystems. We, therefore, call the modular system cost-competitive if at least one of its subsystems is cost-competitive. In addition, the reversibility feature of the system is said to be valuable if both subsystems have positive net present value on their own. The following finding links cost-competitiveness and the value of reversibility to the prevailing market price of hydrogen.
Finding 1: The modular reversible PtG system is cost-competitive if and only if at the prevailing hydrogen market price, p , either \(p\, > \,{p}_{h}^{o}\) or \(p \, < \, {p}_{e}^{o}\) . Reversibility of the modular system is valuable if and only if \(p\in [{p}_{h}^{o},{p}_{e}^{o}]\) .
Figure 2 a illustrates the setting of a modular reversible PtG system that is cost-competitive and for which reversibility is valuable. Note that reversibility of the modular system cannot be of value unless \({p}_{h}^{o}\, < \,{p}_{e}^{o}\) .
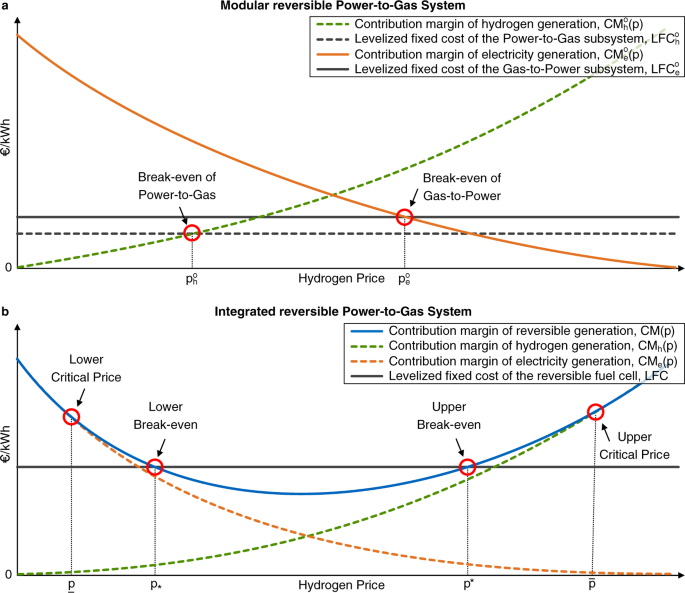
The figure illustrates the potential cost-competitiveness and value of reversible operation in terms of the respective break-even prices of ( a ) a modular reversible Power-to-Gas system, and ( b ) an integrated reversible Power-to-Gas system.
For the integrated reversible PtG system, the LFC of the system is denoted by \(LFC\) . Cost competitiveness of the integrated system then requires that the optimized aggregate contribution margin, C M ( p ), exceeds \(LFC\) . The reversibility of the integrated system is said to be valuable if at the prevailing market price of hydrogen, p , investment in the system is cost-competitive and, furthermore, the system operates in both directions for select hours of the year, i.e., both sets \(\{t| C{F}_{h}^{* }(t| p) \, > \, 0\}\) and \(\{t| C{F}_{e}^{* }(t| p) \, > \, 0\}\) have positive length across the hours of the year.
Figure 2 b illustrates a setting in which the reversibility feature of the integrated reversible PtG system is valuable. We note that when viewed as a function of p , the optimized contribution margin, C M ( ⋅ ), is drawn as a U-shaped curve. This follows directly from the convexity of this function in p (see Methods), combined with the observation that C M ( p ) is increasing for large values of p and again increasing as p becomes small, possibly negative. The U-shape of C M ( ⋅ ) implies that there exist at most two break-even points at which C M ( p ) = LFC. These points are denoted by \({p}_{* }\) and \({p}^{* }\) , respectively.
To examine the value of reversibility, suppose hypothetically that the integrated system could operate in only one direction. For instance, suppose the system is constrained to only produce hydrogen (i.e., C F e in ( 4 ) is set identically equal to zero for all t ). For sufficiently large values of p , there then exists a critical value denoted by \(\bar{p}\) such that \(CM(\bar{p})=C{M}_{h}(\bar{p})\) . This equality holds for all \(p\ge \bar{p}\) . Conversely, there exists a lower critical price below which only electricity generation would be valuable, that is, C M ( p ) = C M e ( p ) for all \(p\le \underline{p}\) .
Finding 2: The integrated reversible PtG system is cost-competitive if and only if the prevailing hydrogen market price, p , does not fall into the range [ \({p}_{* }\) , \({p}^{* }\) ]. Reversibility of the integrated system is valuable if and only if either \(p\in (\underline{p},{p}_{* })\) or \(p\in ({p}^{* },\bar{p})\) .
Finding 2 shows that an integrated reversible PtG system is cost-competitive if the market price of hydrogen moves either into an upper or lower range relative to the price at which the optimized contribution margin reaches its minimum. For the case where \(p\in ({p}^{*},\bar{p})\) , Fig. 2 b depicts the possibility that the integrated system primarily generates hydrogen but also operates bi-directionally. Such systems could create an effective buffer against the intermittency of renewables when power is absorbed from the electricity market for hydrogen conversion, yet occasionally electricity is generated at hours of limited power supply and correspondingly high power prices. The range of hydrogen prices at which an integrated system generates both outputs hinges, in addition to cost, on the round-trip efficiency and the volatility in power prices (Fig. 1) .
An implicit assumption underlying Finding 2 and Fig. 2 b is that LFC exceeds the minimum of the C M ( ⋅ ) curve, for otherwise the break-even prices \({p}_{* }\) and \({p}^{* }\) do not exist (we ignore the non-generic scenario in which there is exactly one break-even price at a tangential point). In case L F C < C M ( ⋅ ) for all p , the integrated reversible PtG system will always be cost-competitive and reversibility will be of value for all hydrogen prices within the interval \((\underline{p},\bar{p})\) . In this case, the flexibility of the integrated reversible PtG system allows it to compensate for any decline in the prevailing market price of hydrogen by turning to electricity production for a larger share of the available time.
Current economics of reversible Power-to-Gas
To apply the preceding model framework, we calibrate the model parameters in the current market environment of Germany and Texas. Both jurisdictions have recently deployed considerable amounts of renewable energy 36 . While Germany has maintained coal and natural gas plants as capacity reserves, Texas has retired several conventional generators 37 . The average wholesale electricity price in 2019 was comparable for both jurisdictions, yet power prices in Texas exhibited much higher volatility. As detailed further in Methods and Supplementary Tables 2 – 5 , our calculations rely on a range of data sources collected from journal articles, industry data, and publicly available reports. Table 1 summarizes the average values of key cost and operational parameter estimates.
Our numbers for the modular PtG system are based on a one-directional PEM electrolyzer and a combined-cycle gas turbine. Recent literature attributes about the same conversion rate to stationary PEM fuel cells as to combined-cycle gas turbines, though the former also entail higher system prices 20 , 38 . For the integrated reversible PtG system, we consider unitized generative SOC fuel cells that are already commercially available 30 , 38 . Regarding the conversion efficiency, we note that PEM electrolyzers attain a near-constant efficiency beyond a small threshold utilization level 30 . For integrated PtG systems, we interpret the conversion efficiency parameters identified in the literature (shown in Table 1) as those obtained at full capacity utilization. Thus far, the existing literature provides no evidence on the shape of the functions η h ( ⋅ ) and η e ( ⋅ ). If these conversion rates were to decrease significantly for capacity utilization values approaching one, our findings regarding the cost competitiveness of integrated reversible PtG systems should be interpreted as a lower bound, because the achievable optimized contribution margins might then be larger for capacity factors strictly less than one. Supplementary Note 2 further examines the sensitivity of our numerical findings to changes in the conversion rates of such systems.
The investing party is assumed to have access to the day-ahead wholesale market for electricity (see Supplementary Note 3 for findings based on the real-time wholesale market for electricity). In order to accelerate the transition towards renewable energy, the German government recently decided that electricity purchases for water electrolysis are exempted from certain taxes and fees paid by industrial customers 39 . In Texas, the investing party is assumed to be able to purchase electricity at wholesale prices subject to a markup, as imposed by suppliers like Griddy (see Supplementary Tables 4 – 5) .
We first determine the hydrogen break-even prices. To assess the cost competitiveness of each (sub-)system, we then compare the break-even prices to prevailing transaction prices for hydrogen supply. These values are applicable benchmarks for hydrogen as both an input and an output when the PtG (or GtP) system can be installed nearby a hydrogen or electricity customer. Market prices currently fall into three segments that vary with purity and scale (volume): large-scale supply between 1.5 and 2.5 €/kg, medium-scale between 3.0 and 4.0 €/kg, and small-scale above 4.0 €/kg 33 .
Our calculations yield break-even prices for the modular electrolyzer ( \({p}_{h}^{o}\) ) of 3.18 €/kg in Germany and 2.98 $/kg in Texas, while the break-even prices for the modular gas turbine ( \({p}_{e}^{o}\) ) are 0.57 €/kg in Germany and 1.31 $/kg in Texas (Table 2 , see Supplementary Tables 6 – 7 for details). The much higher break-even price for the GtP system in Texas must be attributed to the higher volatility in Texas wholesale electricity prices, which in 2019 exceeded 0.15 $¢/kWh on a regular basis.
Finding 1 implies that modular PtG conversion is cost-competitive in both jurisdictions relative to the prices paid for small- and medium-scale hydrogen supply, while the GtP subsystem is not. Furthermore, the reversibility of the modular system cannot be valuable relative to any prevailing market price for hydrogen because \({p}_{h}^{o}\, > \,{p}_{e}^{o}\) in both jurisdictions. Our results here confirm the commonly held view that one-directional GtP systems are currently not economically viable 9 , 19 , 20 .
For the integrated system, our calculations yield break-even prices of 0.03 €/kg for \({p}_{* }\) and 3.38 €/kg for \({p}^{* }\) in Germany, while the break-even prices in Texas are −0.09 $/kg and 2.78 $/kg, respectively (Table 2) . The substantially smaller \({p}^{* }\) in Texas reflects the higher volatility in wholesale power prices. By Finding 2, the integrated system is thus cost-competitive when hydrogen is sold to small- and medium-scale customers in Germany. In Texas, cost competitiveness is achieved even relative to a hydrogen price of at least $2.78 per kg, a value that is borderline for industrial-scale supply.
Regarding the value of reversibility for the integrated system, our calculations yield upper and lower critical prices ( \(\underline{p}\) and \(\bar{p}\) ) of −1.81 €/kg and 2.43 €/kg, respectively, in Germany. In Texas, the corresponding values are 0.59 $/kg for \(\underline{p}\) , while \(\bar{p}\) exceeds 5.0 $/kg. Because the hydrogen prices for medium scale supply fall “comfortably” into the range \(({p}^{* },\bar{p})=(2.78,5.0)\) , we conclude that the reversibility of the integrated PtG system is already valuable in the current Texas environment. Contrary to frequently articulated views in the popular press, the generation of electric power from hydrogen is therefore already economical, provided such generation is part of an integrated PtG system that mainly produces hydrogen yet only occasionally operates in the reverse direction to generate electricity. Such systems can therefore be effective in buffering the increasing volatility in power markets resulting from the growing reliance on intermittent renewable energy sources.
A direct comparison of the modular one-sided and the integrated reversible PtG systems shows that the latter is already positioned more competitively despite its substantially higher systems price, as the break-even price of $2.78 per kg is below the corresponding $2.98 per kg for the modular electrolyzer.
Prospects for reversible Power-to-Gas
Recent technological progress in reversible PtG systems suggests further improvements in terms of declining system prices and increasing conversion efficiencies 40 , 41 , 42 . System prices of PEM electrolyzers are forecast to decline at an annual rate of 4.77%, while conversion rates are likely to increase linearly to on average 0.023 kg/kWh by 2030 20 , 33 , 43 . For combined-cycle gas turbines, both of these parameters are expected to remain unchanged.
To assess the cost dynamics of the unitized generative SOC fuel cell, we rely on a hand-collected data set of N = 79 price observations, as described in Methods. We estimate the trajectory of system price by means of a univariate regression covering the years 2000–2019. The functional form of the regression is a constant elasticity model of the form: v ( i ) = v (0) ⋅ β i , with v ( i ) representing the system price in year i . As shown in Fig. 3 , the resulting estimate for the annual price decline is 8.95% ( β = 0.9105) with a 95% confidence interval of ± 3.20% ( R 2 = 0.27).
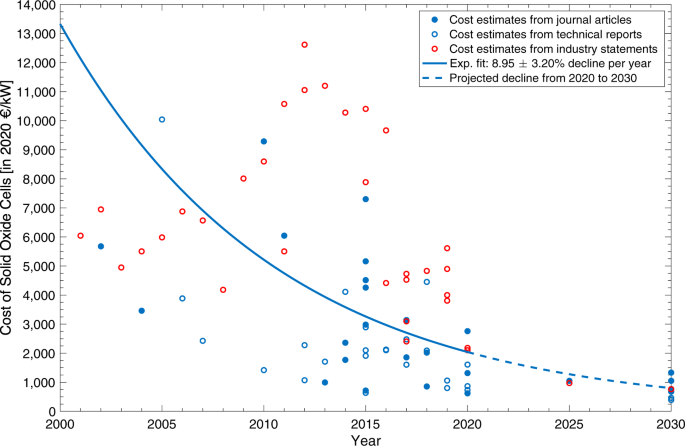
Cost data comes from multiple sources. The univariate regression suggests a constant cost decline over the coming years. The fairly large variance in system prices illustrates the relative novelty of the technology.
The conversion rate of the regenerative SOC fuel cell is expected to increase linearly to on average 0.024 kg/kWh for hydrogen generation and 21.67 kWh/kg for power generation by 2030 20 , 38 . Our calculations are based on the current distribution of power prices to isolate the effects of falling system prices and improved conversion rates. A fall in the average of power prices in connection with rising price volatility, as previous studies suggest 13 , 14 , 44 , 45 , would affect the economics of either system favorably.
Our model results in a trajectory of break-even prices through 2030 as shown in Fig. 4 (see Supplementary Tables 8 , 9 for details). The green lines indicate that the modular electrolyzer is likely to become cost-competitive even relative to the lower prices in the large-scale hydrogen market segment. This conclusion emerges sooner in Texas due to higher volatility in power prices. The break-even prices for the modular gas turbine, as depicted by the orange lines, are projected to remain unchanged. Even though the gap between \({p}_{h}^{o}\) and \({p}_{e}^{o}\) is shrinking, the reversibility feature of the modular system is unlikely to become valuable during the next decade. This stands in contrast to recent ambitions by gas turbine equipment manufacturers 46 , 47 , 48 .
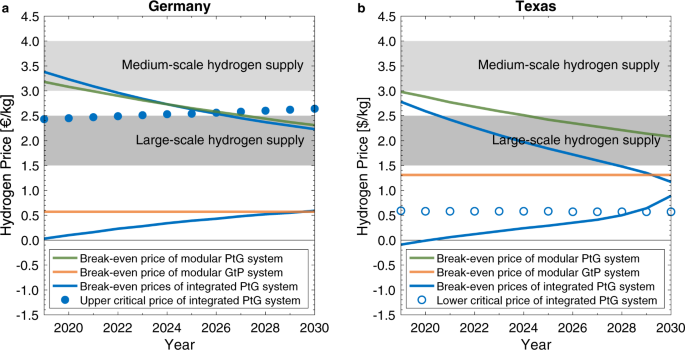
This figure contrasts the relevant hydrogen prices of modular and integrated reversible Power-to-Gas systems in ( a ) Germany and ( b ) Texas with the hydrogen prices attained in different market segments. The lower critical price of the integrated system in Germany is consistently below −1.5 €/kg. The upper critical price of the integrated system in Texas is consistently above 5.0 $/kg.
The integrated system, in contrast, is projected to become widely cost-competitive for large-scale hydrogen supply in both jurisdictions as shown by the upper blue lines in Fig. 4 . We furthermore project the reversibility feature of the integrated system to become increasingly valuable in both jurisdictions as indicated by the falling upper blue lines. In fact, for Texas, the range [ \({p}_{* }\) , \({p}^{* }\) ] is almost closing by the end of the coming decade. As explained in the modeling section, a closing of the range corresponds to the scenario where the flexibility inherent in the unitized regenerative fuel cell allows it to achieve an optimized contribution margin that exceeds the LFC of the system, regardless of the prevailing hydrogen price.
In Germany, the reversibility feature of the integrated system is likely to deliver value starting in the second half of the coming decade. This can be seen in Fig. 4 a by comparing the upper blue line with the blue dots, which illustrate the trajectory of the upper critical prices ( \(\bar{p}\) ) for the integrated system. The reason is that, as the upper break-even price falls, the reversible PtG system will increasingly switch to power generation, as opposed to staying idle, when electricity prices peak (Fig. 1) .
Our analysis has demonstrated that recent advances in unitized regenerative solid oxide fuel cells already make such systems competitive relative to current hydrogen prices. By taking advantage of fluctuations in hourly electricity prices, reversible PtG systems not only act as buffers in electricity markets, they also broaden the supply sources for hydrogen as an industrial input and general energy carrier. If recent trends in the acquisition cost of SOCs continue over the next 5–10 years, our projections indicate that such systems will remain competitive even in the face of substantially lower hydrogen prices, as the electrolyzer then adjusts by operating more frequently as a Gas-to-Power system.
Several promising avenues for future research emerge from our analysis. Earlier work has shown that the economics of electrolyzers can be improved by vertically integrating them with upstream renewable energy sources to achieve operational synergies 49 . It remains to be seen to what extent the addition of a renewable power source would improve the capacity utilization of a reversible PtG system and, therefore, lower the corresponding break-even values. Furthermore, if one views a reversible PtG system as an energy storage device, the natural question is how its competitiveness compares to that of other storage technologies, such as batteries or pumped hydro-power systems 8 , 9 , 50 , 51 .
From an industry and policy perspective, we note that the inherent flexibility of integrated reversible PtG systems makes them valuable during periods of electricity scarcity, including regular demand peaks and irregular supply shocks. With increasing penetration levels of renewable energy, this flexibility feature is likely to become more valuable over time. We finally note that our projections regarding the economic positioning of reversible PtG systems in the future have relied on a regression model that presumes that cost declines are a function of calendar time. Yet, the literature on clean energy technologies has shown that cost declines are not merely an exogenous function of time but instead are determined endogenously by the cumulative number of deployments of these systems 43 . Policy-makers should keep these long-term benefits in mind in adopting regulatory policies aimed at accelerating the rate of PtG system deployments in the short run.
Derivation of the aggregate contribution margins
We begin with the derivation of the optimized aggregate contribution margin, CM ( p ), that is attainable annually if the investor acquires a 1 kW system of the integrated reversible PtG system and the prevailing market price of hydrogen is p . By construction:
subject to 0 ≤ C F h , C F e ≤ 1 and the technical constraint that the unitized regenerative fuel cell can only run in one direction at any point in time. It follows that C M ( p ) is additively separable and can be written as C M ( p ) = C M h ( p ) + C M e ( p ), with:
Here, \(C{F}_{h}^{* }(t| p)\) and \(C{F}_{e}^{* }(t| p)\) are chosen to maximize [ η h ( C F h ) ⋅ p − q ( t ) − w h ] and \([q(t)-\frac{p}{{\eta }_{e}(C{F}_{e})}-{w}_{e}]\) , respectively, at all points in time t .
For the modular reversible PtG systems, the aggregate optimized contribution margins \(C{M}_{h}^{o}(p)\) and \(C{M}_{e}^{o}(p)\) are derived in direct analogy to ( 8 ).
Convexity of C M ( ⋅ ) in p
We demonstrate the convexity of the aggregate annual contribution margin pointwise, that is, convexity holds at any point in time t . Specifically, it suffices to show that for any 0 ≤ λ ≤ 1:
where p λ ≡ λ ⋅ p 1 + (1 − λ ) ⋅ p 0 , \(A(t| p)\equiv {\eta }_{h}(C{F}_{h}^{* }(t| p))\cdot p-q(t)-{w}_{h}\) and \(B(t| p)\equiv q(t)-\frac{p}{{\eta }_{e}(C{F}_{e}^{* }(t| p))}-{w}_{e}\) . As noted above, for any p , either A ( t ∣ p ) ≤ 0 or B ( t ∣ p ) ≤ 0 because η h ( ⋅ ) ⋅ η e ( ⋅ ) ≤ 1.
Suppose now, without loss of generality, that A ( t ∣ p λ ) > 0 in which case the left-hand side of the preceding inequality is equal to A ( t ∣ p λ ). Finally, the right-hand side of the above inequality is given by:
By construction, this last expression is at least as large as λ ⋅ A ( t ∣ p 1 ) + (1 − λ ) ⋅ A ( t ∣ p 0 ), which, because of the linearity of A ( t ∣ ⋅ ) in p , is equal to A ( t ∣ p λ ), thus establishing the desired inequality. The claim regarding convexity of C M ( ⋅ ) then follows from the observation that the sum (integral) of convex functions is also convex.
Net present value of the reversible PtG systems
As before, we focus on integrated reversible PtG systems, with the derivation for modular systems being entirely analogous. The LFC of the system, LFC , aggregates all fixed expenditures required over the life of the reversible PtG system. This aggregate expenditure is then divided by L , the levelization factor that expresses the discounted number of hours that the capacity is available over its lifetime. The resulting cost is then a unit cost per hour of operation. Formally:
Here, f represents the levelized value of fixed operating costs, c represents the levelized capacity cost per kWh, and Δ captures the impact of income taxes and the depreciation tax shield. Denoting by v the system price of the regenerative fuel cell per kW of peak electricity absorption and desorption, we have:
with the levelization factor calculated as:
Here, m denotes the number of hours per year, that is, m = 8760 and the parameter T represents the number of years of useful economic life of the system. Since capacity may degrade over time, we denote by x the degradation factor so that x i −1 gives the fraction of the initial capacity that is functioning in year i . The parameter γ = (1 + r ) −1 and represents the discount factor with r as the cost of capital. This interest rate should be interpreted as the weighted average cost of capital if the levelized cost is to incorporate returns for both equity and debt investors. Similarly, the levelized fixed operating cost per kWh similarly comprises the total discounted fixed operating cost incurred over the lifetime of the system:
The cost of capacity is affected by corporate income taxes through a debt and a depreciation tax shield, as interest payments on debt and depreciation charges reduce the taxable earnings of a firm. The debt tax shield is included in the calculation if r is interpreted as the weighted average cost of capital. Let d i denote the allowable tax depreciation charge in year i . Since the assumed lifetime for tax purposes is usually shorter than the actual economic lifetime, we set d i = 0 in those years. If α represents the effective corporate income tax rate, the tax factor is given by:
The formal claim then is that the net present value of an investment in one kW of the integrated reversible PtG system is given by:
This relation is readily verified by noting that the after-tax cash flows in year i is:
where I i ( p ) denotes the taxable income in year i . Specifically:
Since C F L 0 = − v , the discounted value of all after-tax cash flows is indeed equal to the expression in ( 16 ). Similar reasoning yields that the unit net present values of the modular PtG and GtP systems are, respectively, given by:
Cost dynamics of solid oxide cells
We collected cost estimates from a range of information sources, including industry publications, academic articles in peer-reviewed journals and technical reports by agencies, consultancies, and analysts. These documents were retrieved by searching the database Scopus and the web with Google’s search engine using a combination of one of the five technology-specific keywords ‘reversible electrolyzer’, ‘reversible fuel cell’, ‘solid oxide electrolysis cell’, ‘solid oxide fuel cell’, or ‘reversible PtG’ with the two economic keywords ‘cost’ and ‘investment’. For industry statements, we also searched with the name of a manufacturer in combination with the economic keywords. For the Google search, we reviewed the top 100 search results. The review and the data set is documented in an Excel file available as Supplementary Data 1 .
The review yielded 211 sources, which we filtered by several criteria to ensure quality and timeliness. First, we excluded results published before the year 2000 and, for journal articles, results published in a journal with a rank below 0.5 in the Scimago Journal and Country Rank. The threshold of 0.5 showed to be effective for excluding articles published, for instance, in conference proceedings without peer-review. As for technical reports, we only included results that could convince through appearance, writing, clarity of methodology, and reputation of the author(s) or authoring organization(s). These measures removed 47 sources. Reviewing the resulting stock of sources, we further excluded sources that did not provide direct cost or efficiency data (49) and sources citing other articles as original sources (29). These citations were traced back to the original source. If the original was new, we added it to the pool. We further added sources that we found with a previous review 33 and that were new to the pool.
Our procedure left 86 sources with original data from industry or an original review of multiple sources and yielded 89 cost estimates. In case the sources issued range estimates, we took the arithmetic mean of the highest and the lowest value. The common currency is Euro and all data points in other currencies were converted using the average exchange rate of the respective year as provided by the European Central Bank. Regarding inflation, all historic cost estimates were adjusted using the HCPI of the Euro Zone as provided by the European Central Bank. The cost estimates were winsorized at a 1.0% level. Figure 3 in the main body shows the cost estimates and regression results.
Data availability
The data used in this study are referenced in the main body of the paper and the Supplementary Information. Data that generated the plots in the paper are provided in the Supplementary Information. Additional information is available from the corresponding author upon request.
Code availability
Computational code is available upon request to the corresponding author.
Davis, S. J. et al. Net-zero emissions energy systems. Science 360 , 9793 (2018).
Olauson, J. et al. Net load variability in the Nordic countries with a highly or fully renewable power system. Nat. Energy 1 , 1–14 (2016).
Article Google Scholar
Sternberg, A. et al. Power-to-what?—Environmental assessment of energy storage systems. Energy Environ. Sci. 8 , 389–400 (2015).
Article CAS Google Scholar
Comello, S. & Reichelstein, S. The emergence of cost effective battery storage. Nat. Commun. 10 , 2038 (2019).
Article ADS Google Scholar
Shaner, M. R., Atwater, H. A., Lewis, N. S. & McFarland, E. W. A comparative technoeconomic analysis of renewable hydrogen production using solar energy. Energy Environ. Sci. 9 , 2354–2371 (2016).
Van Vuuren, D. P. et al. Alternative pathways to the 1.5 ∘ C target reduce the need for negative emission technologies. Nat. Clim. Change 8 , 391–397 (2018).
Parkinson, B., Balcombe, P., Speirs, J. F., Hawkes, A. D. & Hellgardt, K. Levelized cost of CO 2 mitigation from hydrogen production routes. Energy Environ. Sci. 12 , 19–40 (2019).
Arbabzadeh, M., Sioshansi, R., Johnson, J. X. & Keoleian, G. A. The role of energy storage in deep decarbonization of electricity production. Nat. Commun. 10 , 3413 (2019).
Sepulveda, N. A., Jenkins, J. D., Edington, A., Mallapragada, D. S. & Lester, R. K. The design space for long-duration energy storage in decarbonized power systems. Nat. Energy 6 , 506–516 (2021).
Hauch, A. et al. Recent advances in solid oxide cell technology for electrolysis. Science 370 , 6513 (2020).
van Renssen, S. The hydrogen solution? Nat. Clim. Change 10 , 799–801 (2020).
Ueckerdt, F. et al. Potential and risks of hydrogen-based e-fuels in climate change mitigation. Nat. Clim. Change 11 , 384–393 (2021).
Wozabal, D., Graf, C. & Hirschmann, D. The effect of intermittent renewables on the electricity price variance. OR Spectr. 38 , 687–709 (2016).
Article MathSciNet Google Scholar
Ketterer, J. C. The impact of wind power generation on the electricity price in Germany. Energy Econ. 44 , 270–280 (2014).
Guerra, O. J. et al. The value of seasonal energy storage technologies for the integration of wind and solar power. Energy Environ. Sci. 13 , 1909–1922 (2020).
Staffell, I. et al. The role of hydrogen and fuel cells in the global energy system. Energy Environ. Sci. 12 , 463–491 (2019).
Guerra, O. J., Eichman, J., Kurtz, J. & Hodge, B. M. Cost competitiveness of electrolytic hydrogen. Joule 3 , 2425–2443 (2019).
Rau, G. H., Willauer, H. D. & Ren, Z. J. The global potential for converting renewable electricity to negative-CO 2 -emissions hydrogen. Nat. Clim. Change 8 , 621–626 (2018).
Braff, W. A., Mueller, J. M. & Trancik, J. E. Value of storage technologies for wind and solar energy. Nat. Clim. Change 6 , 964–969 (2016).
International Energy Agency. The Future of Hydrogen . Tech. Rep. (International Energy Agency, 2019).
Ding, H. et al. Self-sustainable protonic ceramic electrochemical cells using a triple conducting electrode for hydrogen and power production. Nat. Commun. 11 , 1907 (2020).
Regmi, Y. N. et al. A low temperature unitized regenerative fuel cell realizing 60% round trip efficiency and 10,000 cycles of durability for energy storage applications. Energy Environ. Sci. 13 , 2096–2105 (2020).
Elcogen. Reversible solid oxide cell technology as a power storing solution for renewable energy (Italy). http://bit.ly/385mR4N (2018).
Schmidt, O., Melchior, S., Hawkes, A. & Staffell, I. Projecting the future levelized cost of electricity storage technologies. Joule 13 , 1–20 (2019).
Proost, J. State-of-the art CAPEX data for water electrolysers, and their impact on renewable hydrogen price settings. Int. J. Hydrog. Energy 44 , 4406–4413 (2019).
Pellow, M. A., Emmott, C. J., Barnhart, C. J. & Benson, S. M. Hydrogen or batteries for grid storage? A net energy analysis. Energy Environ. Sci. 8 , 1938–1952 (2015).
Mogensen, M. B. et al. Reversible solid-oxide cells for clean and sustainable energy. Clean. Energy 3 , 175–201 (2019).
Peng, X. et al. Hierarchical electrode design of highly efficient and stable unitized regenerative fuel cells (URFCs) for long-term energy storage. Energy Environ. Sci. 13 , 4872–4881 (2020).
International Energy Agency. Hydrogen Projects Database (2020). https://www.iea.org/data-and-statistics/data-product/hydrogen-projects-database . Date accessed: 03.04.2022.
Buttler, A. & Spliethoff, H. Current status of water electrolysis for energy storage, grid balancing and sector coupling via power-to-gas and power-to-liquids: A review. Renew. Sustain. Energy Rev. 82 , 2440–2454 (2018).
Beney, A. M. Investigation into the Heat Up Time for Solid Oxide Fuel Cells in Automotive Applications . Tech. Rep. (University of Guelph, 2018).
Graves, C., Ebbesen, S. D., Mogensen, M. & Lackner, K. S. Sustainable hydrocarbon fuels by recycling CO 2 and H 2 O with renewable or nuclear energy. Renew. Sustain. Energy Rev. 15 , 1–23 (2011).
Glenk, G. & Reichelstein, S. Economics of converting renewable power to hydrogen. Nat. Energy 4 , 216–222 (2019).
Article ADS CAS Google Scholar
Reichelstein, S. & Rohlfing-Bastian, A. Levelized product cost: Concept and decision relevance. Account. Rev. 90 , 1653–1682 (2015).
MIT. The Future of Coal: Options for a Carbon-Constrained World . Tech. Rep. (Massachusetts Institute of Technology, 2007).
International Energy Agency. C O 2 Emissions from Fuel Combustion 2017 - Highlights . Tech. Rep. (International Energy Agency, 2017).
Reuters. Texas power prices jump to record high as heat bakes state. https://reut.rs/2tw4h78 (2019).
Weidner, E., Ortiz Cebolla, R. & Davies, J. Global Deployment of Large Capacity Stationary Fuel Cells. Tech. Rep. (Publications Office of the European Union, 2019).
Bundesrat. 383/19 Gesetz zur Änderung des Gesetzes über Energiedienstleistungen und andere Energieeffizienzmaßnahmen. https://www.bundesrat.de/bv.html?id=0383-19 (2019).
Rivera-Tinoco, R., Schoots, K. & Van Der Zwaan, B. Learning curves for solid oxide fuel cells. Energy Convers. Manag. 57 , 86–96 (2012).
Wei, M., Smith, S. J. & Sohn, M. D. Experience curve development and cost reduction disaggregation for fuel cell markets in Japan and the US. Appl. Energy 191 , 346–357 (2017).
Saba, S. M., Müller, M., Robinius, M. & Stolten, D. The investment costs of electrolysis—A comparison of cost studies from the past 30 years. Int. J. Hydrog. Energy 43 , 1209–1223 (2018).
Glenk, G., Meier, R. & Reichelstein, S. Cost dynamics of clean energy technologies. Schmalenbach J. Bus. Res. 73 , 179–206 (2021).
Paraschiv, F., Erni, D. & Pietsch, R. The impact of renewable energies on EEX day-ahead electricity prices. Energy Policy 73 , 196–210 (2014).
Kök, A. G., Shang, K. & Yücel, S. Impact of electricity pricing policies on renewable energy investments and carbon emissions. Manag. Sci. 64 , 131–148 (2018).
Uniper SE. Siemens und Uniper bündeln Kräfte bei Dekarbonisierung der Stromerzeugung. https://bit.ly/3iuJ0lm (2020).
Businesswire. Mitsubishi power cuts through the complexity of decarbonization: Offers the world’s first green hydrogen standard packages for power balancing and energy storage (2020). https://bwnews.pr/3yXxMLT .
General Electric. Hydrogen for Power Generation . Tech. Rep. (General Electric, 2021).
Glenk, G. & Reichelstein, S. Synergistic value in vertically integrated Power-to-Gas energy systems. Prod. Oper. Manag. 29 , 526–546 (2020).
Albertus, P., Manser, J. S. & Litzelman, S. Long-duration electricity storage applications, economics, and technologies. Joule 4 , 21–32 (2020).
Baumgarte, F., Glenk, G. & Rieger, A. Business models and profitability of energy storage. iScience 23 , 101554 (2020).
Download references
Acknowledgements
We gratefully acknowledge financial support through the Deutsche Forschungsgemeinschaft (DFG, German Research Foundation): Project-ID 403041268, TRR 266. This research was also supported by the Joachim Herz Stiftung and the Hanns-Seidel-Stiftung with funds from the Federal Ministry of Education and Research of Germany. Helpful comments were provided by Stefanie Burgahn, Céleste Chevalier, Stephen Comello, Gunther Friedl, Rebecca Meier, Christian Stoll, Nikolas Wölfing, and colleagues at the University of Mannheim, the Technical University of Munich, and the Massachusetts Institute of Technology. Finally, we thank Lisa Fuhrmann for providing valuable assistance with the data collection.
Open Access funding enabled and organized by Projekt DEAL.
Author information
Authors and affiliations.
Mannheim Institute for Sustainable Energy Studies, University of Mannheim, MIT CEEPR, Massachusetts Institute of Technology, Cambridge, MA, USA
Gunther Glenk
Mannheim Institute for Sustainable Energy Studies, University of Mannheim, Graduate School of Business, Stanford University, Leibniz Centre for European Economic Research (ZEW), Mannheim, Germany
- Stefan Reichelstein
You can also search for this author in PubMed Google Scholar
Contributions
G.G. initiated the research question and the techno-economic model. He also conducted the literature review, the data collection, and the numerical calculations. G.G. and S.R. jointly analyzed the economic model and both contributed to the writing of the paper.
Corresponding author
Correspondence to Gunther Glenk .
Ethics declarations
Competing interests.
The authors declare no competing interests.
Peer review
Peer review information.
Nature Communications thanks Joris Proost and the other, anonymous, reviewer(s) for their contribution to the peer review of this work. Peer reviewer reports are available.
Additional information
Publisher’s note Springer Nature remains neutral with regard to jurisdictional claims in published maps and institutional affiliations.
Supplementary information
Supplementary information, peer review file, description of additional supplementary files, supplementary data 1, rights and permissions.
Open Access This article is licensed under a Creative Commons Attribution 4.0 International License, which permits use, sharing, adaptation, distribution and reproduction in any medium or format, as long as you give appropriate credit to the original author(s) and the source, provide a link to the Creative Commons license, and indicate if changes were made. The images or other third party material in this article are included in the article’s Creative Commons license, unless indicated otherwise in a credit line to the material. If material is not included in the article’s Creative Commons license and your intended use is not permitted by statutory regulation or exceeds the permitted use, you will need to obtain permission directly from the copyright holder. To view a copy of this license, visit http://creativecommons.org/licenses/by/4.0/ .
Reprints and permissions
About this article
Cite this article.
Glenk, G., Reichelstein, S. Reversible Power-to-Gas systems for energy conversion and storage. Nat Commun 13 , 2010 (2022). https://doi.org/10.1038/s41467-022-29520-0
Download citation
Received : 18 February 2021
Accepted : 14 March 2022
Published : 19 April 2022
DOI : https://doi.org/10.1038/s41467-022-29520-0
Share this article
Anyone you share the following link with will be able to read this content:
Sorry, a shareable link is not currently available for this article.
Provided by the Springer Nature SharedIt content-sharing initiative
This article is cited by
Complementary probes for the electrochemical interface.
- Ernest Pastor
- F. Pelayo García de Arquer
Nature Reviews Chemistry (2024)
Applications of the levelized cost concept
- Gunther Friedl
- Lukas Kemmer
Journal of Business Economics (2023)
By submitting a comment you agree to abide by our Terms and Community Guidelines . If you find something abusive or that does not comply with our terms or guidelines please flag it as inappropriate.
Quick links
- Explore articles by subject
- Guide to authors
- Editorial policies
Sign up for the Nature Briefing newsletter — what matters in science, free to your inbox daily.

Pumped Storage Hydropower
Pumped storage hydropower does not calculate LCOE or LCOS, so do not use financial assumptions. Therefore all parameters are the same for the R&D and Markets & Policies Financials cases.
2023 ATB data for pumped storage hydropower (PSH) are shown above. Base Year capital costs and resource characterizations are taken from a national closed-loop PSH resource assessment completed under the U.S. Department of Energy (DOE) HydroWIRES Project D1: Improving Hydropower and PSH Representations in Capacity Expansion Models. Resource assessment and cost assumptions are documented by (Rosenlieb et al., 2022) and subsequent updates are described on NREL's resource data web page: " Closed-Loop Pumped Storage Hydropower Supply Curves ." The ATB considers only closed-loop systems due to their lower environmental impacts: open-loop and other configurations are not included in these estimates. Operation and maintenance (O&M) costs and round-trip efficiency are based on estimates for a 1,000-MW system reported in the 2020 DOE " Grid Energy Storage Technology Cost and Performance Assessment ." (Mongird et al., 2020) . Projected changes in capital costs are based on the DOE Hydropower Vision study (DOE, 2016) and assume different degrees of technology improvement and technological learning.
The three scenarios for technology innovation are:
- Conservative Technology Innovation Scenario (Conservative Scenario): no change from baseline CAPEX and O&M costs through 2050
- Moderate Technology Innovation Scenario (Moderate Scenario): no change from baseline CAPEX and O&M costs through 2050, consistent with the Reference case in the DOE Hydropower Vision study (DOE, 2016)
- Advanced Technology Innovation Scenario (Advanced Scenario): CAPEX reductions of 12% by 2050 based on improved process and design improvements along with advanced manufacturing, new materials, and other technology improvements, consistent with Advanced Technology in the DOE Hydropower Vision study (DOE, 2016) ; no changes to O&M.
Resource Categorization
Resource categorization from a national closed-loop PSH resource assessment is described in detail by (Rosenlieb et al., 2022) with subsequent updates described on NREL's resource data web page: " Closed-Loop Pumped Storage Hydropower Supply Curves ." Individual sites are identified using geospatial algorithms to delineate potential reservoir boundaries, exclude reservoirs that violate technical potential criteria (e.g., protected land, critical habitat), find all possible reservoir pairings, and then eliminate overlapping reservoirs to produce the least-cost set of nonoverlapping reservoir pairs. This procedure is done for alternative storage durations of 8, 10, and 12 hours. Underlying data are site-specific, but for the ATB, resource classes are binned by capital cost such that each class contains a roughly equal amount of total national PSH capacity potential. Binning is done at the national level for the data tables below, and other representations use region-specific cost bins to better represent the distribution of site characteristics in each region. Physical characteristics and capital cost statistics for each ATB class and a 10-hour storage duration are included in the table below.
Resource Class Capacity and Capital Costs
Resource Class Design Values
Scenario Descriptions
Cost reductions in the Advanced Scenario reflect various types of technology innovations that could be applied to PSH facilities. These potential innovations, which are discussed in the DOE Hydropower Vision Roadmap (DOE, 2016) , are largely similar to technology pathways for hydropower without pumping.
Summary of Technology Innovation: Advanced Scenario
Scenario Assumptions
No explicit deployment assumptions or learning rates are used to define the Advanced Technology Innovation Scenario for PSH. All cost reductions are attributed to improved technology, processes, designs, and contracting along with advanced materials and improved construction practices. Deployed PSH capacity is 23 GW in the base year (2021), and the rate of cost reduction is 0.6 %/yr through 2035 and 0.2%/yr from 2035 to 2050.
Representative Technology
The resource assessment procedure requires several design specifications to be defined up front, and for the resource included in the ATB, these include hydraulic heads of 200 m–750 m, a maximum reservoir distance of 12 times the head height, and dam heights of 40 m, 60 m, 80 m, or 100 m (Rosenlieb et al., 2022) and " Closed-Loop Pumped Storage Hydropower Supply Curves " (NREL). Upper and lower reservoir volumes are also assumed to be within 10% of each other. Given the resulting technical specifications of each reservoir pair, the powerhouse (turbine, generator, and electrical equipment) can be sized flexibly for a given reservoir pair, and here data are included for a powerhouse sized to result in 8, 10, or 12 hours of storage duration (i.e., the maximum number of hours generating at rated capacity).
Methodology
This section describes the methodology to develop assumptions for CAPEX, O&M, and round-trip efficiency.
Capital Expenditures (CAPEX)
Capital costs are first calculated for each site using the PSH cost model from Australia National University (Blakers et al., 2019) , adjusted to use a 33% project contingency factor instead of the base 20% assumption to better align with other technologies and U.S. industry practice. The cost model uses reservoir and powerhouse characteristics as inputs to generalized equations for PSH overnight capital cost. These raw costs are then further calibrated to more closely match hydropower industry expectations by multiplying site costs by a factor equal to the ratio of the central CAPEX estimate in (Mongird et al., 2020) for a 1,000-MW, 10-hour facility to the median CAPEX of all sites in the capacity range of 900–1,100 MW (Mongird et al., 2020) . This factor is equal to 1.51, and due to the limited amount of available cost data, this factor is applied uniformly to all sites. Grid connection costs are then added based on the distance from the powerhouse location (assumed at the lower reservoir) to the nearest high-voltage transmission line node (Maclaurin et al., 2021) . Cost assessment is described in detail by (Rosenlieb et al., 2022) .
The maps below plot the median CAPEX in each state for each of the 15 resource classes when individual sites are binned by cost separately for each state. Some states have zero sites identified, largely due to insufficient elevation differences to meet the 200-m minimum head height criteria. The ratio of water conveyance length between reservoirs to head height (L/H ratio) is also shown for individual sites. The display also includes links to a bar chart and a tabular display. The bar chart shows more granular data for each balancing area defined in the Regional Energy Deployment System ( ReEDS ) capacity expansion model (Ho et al., 2021) along with the state average PSH capital cost. The table allows the data to be filtered by class and balancing area to view region- or class-specific data.
Regional PSH Capital Cost by Class
Operation and Maintenance (O&M) Costs
(Mongird et al., 2020) characterize PSH O&M costs using a literature review of recently published sources of PSH cost and performance data. For the 2023 ATB, we use cost estimates for a 1,000-MW plant, which has lower labor costs per power output capacity compared to a smaller facility. O&M costs also include component costs for standard maintenance, refurbishment, and repair. O&M cost reductions are not projected for future years because the relevant technical components are assumed to be mature, so they are constant and identical across all scenarios.
Round-Trip Efficiency
Round-trip efficiency is also based on a literature review by (Mongird et al., 2020) , who report a range of 70%–87% across several sources. The value of 80% is taken as a central estimate, and no improvements are projected either in (Mongird et al., 2020) or here because the relevant technical components are assumed to be mature. Thus, round-trip efficiency is constant and identical across all scenarios.
The following references are specific to this page; for all references in this ATB, see References .
Rosenlieb, Evan, Donna Heimiller, and Stuart Cohen. “Closed-Loop Pumped Storage Hydropower Resource Assessment for the United States.” Golden, CO: National Renewable Energy Laboratory, 2022. https://doi.org/10.2172/1870821 .
Mongird, Kendall, Vilayanur Viswanathan, Jan Alam, Charlie Vartanian, Vincent Sprenkle, and Richard Baxter. “2020 Grid Energy Storage Technology Cost and Performance Assessment.” Washington, D.C.: U.S. Department of Energy, December 2020. https://www.energy.gov/energy-storage-grand-challenge/downloads/2020-grid-energy-storage-technology-cost-and-performance .
DOE. “Hydropower Vision: A New Chapter for America’s Renewable Electricity Source.” Washington, D.C.: U.S. Department of Energy, 2016. https://doi.org/10.2172/1502612 .
Blakers, Andrew, Matthew Stocks, Bin Lu, Kirsten Anderson, and Anna Nadolny. “Global Pumped Hydro Atlas.” Australian National University, 2019. http://re100.eng.anu.edu.au/research/phes /.
Maclaurin, Galen, Nicholas Grue, Anthony Lopez, Donna Heimiller, Michael Rossol, Grant Buster, and Travis Williams. “The Renewable Energy Potential (ReV) Model: A Geospatial Platform for Technical Potential and Supply Curve Modeling.” Golden, CO: National Renewable Energy Laboratory, 2021. https://doi.org/10.2172/1563140 .
Ho, Jonathan, Jonathon Becker, Maxwell Brown, Patrick Brown, Ilya (ORCID:0000000284917814) Chernyakhovskiy, Stuart Cohen, Wesley (ORCID:000000029194065X) Cole, et al. “Regional Energy Deployment System (ReEDS) Model Documentation: Version 2020.” Golden, CO: National Renewable Energy Laboratory, June 9, 2021. https://doi.org/10.2172/1788425 .

IMAGES
VIDEO
COMMENTS
The round trip efficiency (RTE), also known as AC/AC efficiency, refers to the ratio between the energy supplied to the storage system (measured in MWh) and the energy retrieved from it (also measured in MWh). This efficiency is expressed as a percentage (%). The round trip efficiency is a crucial factor in determining the effectiveness of ...
Round-Trip Efficiency. Round-trip efficiency is the ratio of useful energy output to useful energy input. (Mongird et al., 2020) identified 86% as a representative round-trip efficiency, and the 2022 ATB adopts this value. In the same report, testing showed 83-87%, literature range of 77-98%, and a projected increase to 88% in 2030. ...
Round-trip efficiency is a measure of the amount of energy put into a system compared to the amount dispatched, and is expressed as a percentage. A system with a high RTE (75%+) is able to ...
Round-trip efficiency is the percentage of electricity put into storage that is later retrieved. The higher the round-trip efficiency, the less energy is lost in the storage process. According to data from the U.S. Energy Information Administration (EIA), in 2019, the U.S. utility-scale battery fleet operated with an average monthly round-trip ...
Round-Trip Efficiency. Round-trip efficiency is the ratio of useful energy output to useful energy input. (Mongird et al., 2020) identified 86% as a representative round-trip efficiency, and the 2021 ATB adopts this value. References . The following references are specific to this page; for all references in this ATB, see References.
The round-trip energy efficiency of GES system is calculated by dividing the energy output (turbine mode) by the energy input (pumping mode). The round-trip efficiency ranges generally between 70 % and 80 %. It takes values inferior to 100 % due to the different losses occurring within the system. The objective of this study is to assess the ...
provide energy or ancillary services to the grid at any given time. • Round-trip efficiency, measured as a percentage, is a ratio of the energy charged to the battery to the energy discharged from the battery. It can represent the total DC-DC or AC-AC efficiency of the battery system, including losses from self-discharge and other
Round-Trip Efficiency. Round-trip efficiency is the ratio of useful energy output to useful energy input. (Mongird et al., 2020) identified 86% as a representative round-trip efficiency, and the 2021 ATB adopts this value. References . The following references are specific to this page; for all references in this ATB, see References.
Recent times have witnessed significant progress in battery technology due to the growing demand for energy storage systems in various applications. Consequently, battery efficiency has become a crucial aspect of modern battery technology since it directly influences battery performance and lifespan. To guarantee the optimal performance and longevity of batteries, it is essential to measure ...
Enhancing the round-trip efficiency of grid-scale Li-air batteries opens a path to convert low-grade waste heat into valuable electrical energy. ... comparison between the effect of current density on energy efficiency of the hybrid Li-air cells cycling at room temperature and an elevated temperature of 50 °C, and the simplified model used to ...
Efficiencies of all energy conversion steps in this cycle are combined in the metric called round-trip efficiency, which essentially indicates the percentage of energy delivered by the storage system compared to the energy initially supplied to the storage system. The obvious goal is to minimize the conversion losses and thus maximize the ...
The combined system exhibited capacity fade rate <0.07% per day, round-trip energy efficiency >85% and approximately 99% Coulombic efficiency during stable operation for over a week. Cost analysis ...
This paper documents the investigation into determining the round trip energy efficiency of a 2MW Lithium-titanate battery energy storage system based in Willenhall (UK). This research covers the battery and overall system efficiency as well as an assessment of the auxiliary power consumption of the system. The results of this analysis can be used to run the system at its optimal operating ...
The battery passport is proposed as a method to make the use and remaining value of batteries more transparent. The future EU Battery Directive requests this passport to contain the round-trip energy efficiency and its fade. In this paper, an algorithm is presented and demonstrated that estimates the round-trip energy efficiency of a battery pack. The algorithm identifies round trips based on ...
Assuming N = 365 charging/discharging events, a 10-year useful life of the energy storage component, a 5% cost of capital, a 5% round-trip efficiency loss, and a battery storage capacity ...
The round trip efficiency (RTE) of an energy storage system is defined as the ratio of the total energy output by the system to the total energy input to the system, as measured at the point of connection. The RTE varies widely for different storage technologies. A high value means that the incurred losses are low.
Stationary energy storage systems that can operate for many cycles, at high power, with high round-trip energy efficiency, and at low cost are required. Existing energy storage technologies cannot ...
Round-Trip Efficiency. Round-trip efficiency is the ratio of useful energy output to useful energy input. (Cole and Karmakar, 2023) identified 85% as a representative round-trip efficiency, and the 2023 ATB adopts this value. References . The following references are specific to this page; for all references in this ATB, see References.
Image: Duke Energy. Round-trip efficiency of alternative storage technologies is the standout metric for assessing their potential versus lithium-ion, Energy-Storage.news has heard. At last month's RE+ national clean energy industry event, two US-based engineering, procurement and construction (EPC) companies offered their views after ...
The round-trip efficiency for each pathway is calculated by comparing the energy demand that each individual technology requires along the pathways and the usable electric energy that is provided at the end of the pathways. The most efficient pathway for ammonia turns out to be a combination of a decomposition-unit and a solid-oxide fuel cell ...
A low temperature unitized regenerative fuel cell realizing 60% round trip efficiency and 10,000 cycles of durability for energy storage applications. Energy Environ. Sci. 13 , 2096-2105 (2020).
NASA Glenn Research Center (GRC) has recently demonstrated a Polymer Electrolyte Membrane (PEM) based hydrogen/oxygen regenerative fuel cell system (RFCS) that operated for a charge/discharge cycle with round trip efficiency (RTE) greater than 50 percent. The regenerative fuel cell system (RFCS) demonstrated closed loop energy storage over a pressure range of 90 to 190 psig.
Round-Trip Efficiency Round-trip efficiency is also based on a literature review by (Mongird et al., 2020) , who report a range of 70%-87% across several sources. The value of 80% is taken as a central estimate, and no improvements are projected either in (Mongird et al., 2020) or here because the relevant technical components are assumed to ...
Round-trip efficiency is also based on a literature review by (Mongird et al., 2020), who report a range of 70%-87% across several sources. The value of 80% is taken as a central estimate, and no improvements are projected either in (Mongird et al., 2020) or here because the relevant technical components are assumed to be mature.